Seawater to resource technologies with NASICON solid electrolyte: a review
- 1School of Energy and Chemical Engineering, Ulsan National Institute of Science and Technology (UNIST), Ulsan, Republic of Korea
- 2R&D Center, 4TOONE Corporation, Ulsan, Republic of Korea
Seawater represents an inexhaustible reservoir of valuable resources, containing vast quantities of both water and minerals. However, the presence of various impurities in seawater hinders its direct utilization for resource extraction. To address this challenge, an electrochemical method employing a solid electrolyte known as NASICON (Sodium Super Ionic Conductor) offers effective solutions for extracting valuable resources from seawater. The NASICON ceramic acts as a robust barrier against impurities and facilitates the selective transport of Na+. This review provides a comprehensive examination of NASICON ceramics, offering an overview of the concept and highlighting the competitive advantages of NASICON-based electrochemical systems, particularly in the realms of energy storage, hydrogen production, sodium hydroxide and chlorine synthesis, water treatment, and mineral extraction. Furthermore, this study outlines the key challenges that need to be addressed and discusses the trajectory of its development toward becoming a mature technology.
1 Introduction
Earth’s oceans and other saline bodies of water constitute the planet’s most abundant water and mineral resources (Bardi, 2010; Diallo et al., 2015; Loganathan et al., 2017). Encompassing nearly three-quarters of the Earth’s surface (Mero, 1964), they contain approximately 1.371 × 1018 tons of water (Webb, 2021). To comprehend the magnitude of this vast resource, refer to Figure 1A, where a representation of a seawater sphere illustrates the proportion of Earth’s water about the planet’s size. This substantial seawater sphere primarily consists of water (96.56%), with the remaining fraction comprising dissolved salts (3.44%) (Casey, 2018). The predominant ions in these salts, constituting 85.4% of seawater’s salt composition, are Na+ and Cl−, followed by SO42-, Mg2+, Ca2+, K+, Sr2+, HCO3-, Br−, BO32-, and F−.
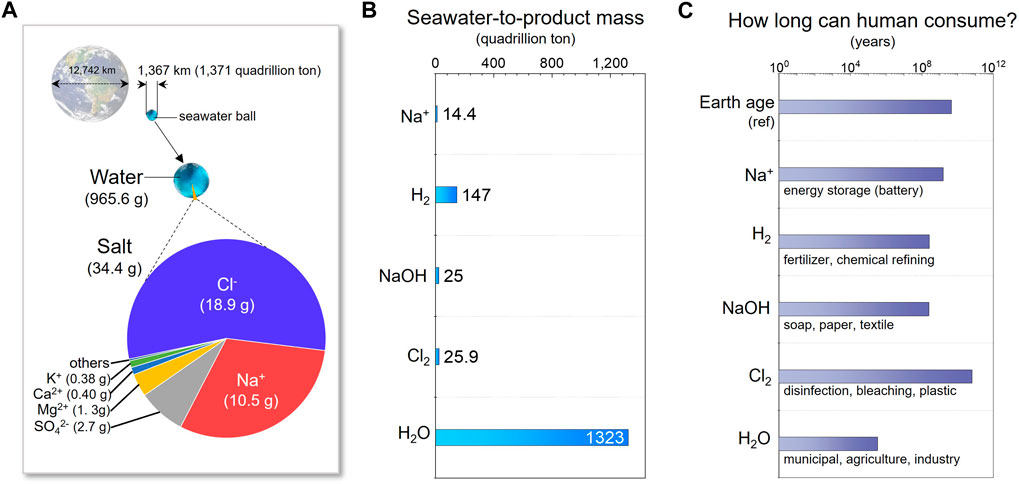
FIGURE 1. (A) Comparison of seawater quantity and size in relation to the Earth, along with a pie diagram displaying seawater components. (B) The estimated total mass of potential products derived from seawater. (C) Estimated time duration for human usage based on annual global total consumption.
Seawater, primarily composed of water and NaCl salts, holds the potential to yield valuable products through electrolytic or electrochemical separation processes. Among the primary products are Na ions (Na+), which function as charge carriers in energy storage devices (Slater et al., 2013; Hwang et al., 2017; Delmas, 2018). While conventional lithium-ion battery (LIB) development may advance through science and technology, challenges with the availability of lithium in the Earth’s crust will likely lead to a substantial price increase (Martin et al., 2017). This would limit LIBs primarily to mobile applications such as electronics and vehicles. Due to the abundant and cost-effective availability of Na+, sodium batteries are viewed as a promising solution for larger-scale stationary applications like energy storage systems (Delmas, 2018).
In addition, hydrogen gas is an emerging clean energy source (Momirlan and Veziroglu, 2002; Rosen and Koohi-Fayegh, 2016), while NaOH and Cl2 bear significant industrial importance (Marleen Pauwels, 2023), and freshwater (H2O) is essential for human survival (Figure 1B) (Ritchie and Roser, 2017). Though the quantities of these products, except freshwater, may appear relatively small, their potential could sustain human consumption for over three hundred thousand years (Figure 1C) (Ritchie and Roser, 2017; IEA, 2020; Jayanthan, 2022; GlobeNewswire, 2023; Kabir, 2023; Statista, 2023). Consequently, harnessing seawater through electrochemical methods represents a promising and sustainable solution for human civilization.
Nevertheless, the presence of various impurities in seawater poses challenges to its cost-effective and energy-efficient utilization. For instance, the conventional chlor-alkali (CA) membrane cell system for NaOH and Cl2 production relies on NaCl solutions, while avoiding the presence of Mg2+ and Ca2+ ions, as they contribute to detrimental scale formation on the membrane in an alkaline environment (O'Brien et al., 2005). Consequently, the direct use of seawater in the CA cell not only yields impure chemicals unsuitable for sale but also compromises the sustainable operation of the production system, resulting in high capital and operational expenses. Similarly, proton exchange membrane (PEM) electrolysis systems for H2 production face similar challenges, requiring high-purity water (resistivity >1 M·Ω·cm, sodium and chloride content <5 μg·L−1) for long-term stability (Khan et al., 2021).
Given these challenges, the selective extraction of desired components from seawater becomes paramount. Various purification and separation processes, including distillation (e.g., multi-effect and multi-stage distillation) (Panagopoulos et al., 2019; Panagopoulos, 2020), reverse osmosis (Kucera, 2019), electrodialysis (Reig et al., 2014; Zhang et al., 2017; Selvaraj et al., 2018), ion exchange (Reig et al., 2014), and chemical processes (Ronquim et al., 2020; Alsabbagh et al., 2021), can be applied. However, these processes often struggle to selectively extract individual target ions. Therefore, a thoughtful combination of multiple processes is necessary to achieve the desired selectivity. The excessive use of these processes, both in terms of energy and capital costs, can severely impede the economic feasibility of seawater utilization (Panagopoulos, 2021).
The primary objective of this article is to review several systems specializing in utilizing Na super ionic conductors (NASICON) for specific resource production. In contrast to seawater-based electrochemical processes coupled with conventional purification and separation methods, direct seawater electrochemical processes employing NASICON solid electrolytes have emerged as efficient seawater-to-resource technologies (SRTs) (Hwang et al., 2019; Sharma et al., 2021; Kim et al., 2022b). Before delving into various NASICON-based SRTs, this study examines the characteristics of NASICON and its compatibility with seawater to meet the requirements for sustainable system operation. Furthermore, this study compares NASICON-based SRTs with conventional seawater-based resource production processes from economic and energy consumption perspectives. These NASICON-based technologies enable the direct use of seawater for various functions, including energy storage, hydrogen storage and production, sodium hydroxide and chlorine generation, freshwater (desalination) production, all without the need for additional refinement or purification stages.
2 Principle of NASICON-based seawater electrolytic processes
The fundamental principle underlying NASICON-based Electrolytic Seawater-to-Resource Technologies (SRTs) is the selective exclusion of most components present in seawater, allowing only the passage of Na+ (Figure 2A). The NASICON solid electrolyte serves as a ceramic-based separator, enabling the exclusive transport of Na+, whose mechanism is detailed in Section 3.1. Consequently, NASICON facilitates sustainable redox reactions in seawater, encompassing Na+ redox reactions, water electrolysis, and chloride oxidation, while inhibiting undesirable parasitic electrochemical and chemical reactions.
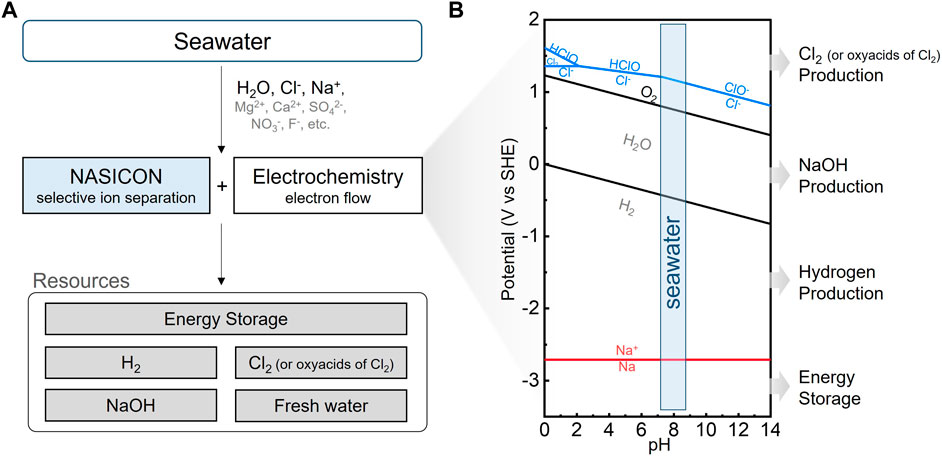
FIGURE 2. (A) Schematic flowchart illustrating electrochemical seawater-to-resource technologies utilizing NASICON. (B) Simulated Pourbaix diagram for seawater at 25°C, with assumed activity coefficients for all species to be 1.
This section offers an overview of the primary mechanisms within each SRT and summarizes the key electrochemical reactions associated with their respective functions (Figure 2B). The following reactions occur in seawater environments and systems. In Section 3.2, the first NASICON-based SRT focuses on energy storage by utilizing Na+ as the charge carrier. It employs Na+ from seawater as a charge carrier and converts electrical energy into chemical energy through various redox reactions at the negative electrode such as Na deposition/dissolution (Eq. 1), alloying/dealloying, or intercalation/deintercalation reactions. Oxygen evolution and reduction reactions typically take place at the positive electrode (Eq. 3). This system utilizes abundant seawater and air as open cathodes, enhancing its economic feasibility by eliminating the need for a conventional positive electrode.
Section 3.3 introduces the second NASICON-based SRT, specializing in H2 storage and production. It leverages the Na metal deposition/dissolution reaction (Eq. 1) for extracting H2 from seawater, akin to alkali-metal-mediated H2 storage processes. In this system, H2 is produced via an electrolytic water reaction (Eq. 2, right direction) during the Na metal dissolution. Due to the remarkable volumetric capacity of Na metal for H2 storage at room temperature and atmospheric pressure, it presents a promising alternative to conventional hydrogen storage methods.
In Section 3.4, the subsequent NASICON-based SRT demonstrates NaOH production using extracted Na+ from seawater. This technology shares similarities with NASICON-based SRT for hydrogen storage and production since both systems utilize Na+ from seawater. Hydroxide production involves an electrolytic water reaction (Eq. 3, right direction) at the positive electrode, while Na+ was continuously supplied from the anode (or anolyte) side. This direct seawater electrolytic process offers economic advantages over conventional hydroxide production methods using seawater.
Section 3.5 explores Cl2 and oxyacids of chlorine (e.g., ClO−, HClO, etc.) production using chloride ions in seawater at the anode. NASICON-based SRTs utilize various dominant chloride-ion-based electrochemical reactions, dependent on the pH of the solution, for applications like Cl2 production and disinfection. Compared with conventional Cl2 (or oxyacids of chlorine) production technologies, this system exhibits superior energy efficiency in chemical production owing to NASICON’s effective prevention of production loss attributed to the reduction of oxidized Cl2(or oxyacids of chlorine) species.
Finally, Section 3.6 delves into the electrochemical desalination process using the NASICON software. This system employs NASICON as a cation exchange membrane (CEM) to separate cations from water. Due to its high selectivity for Na+, this system yields a highly purified NaCl solution as a concentrated stream, unlike the high concentrations of waste brine conventionally produced in reverse osmosis seawater desalination processes. This property of NASICON-based SRT enables sustainable and cost-effective desalination.
3 NASICON based seawater-to-resource technologies
3.1 NASICON ceramic membrane
The stringent requirements for membranes in Seawater-to-Resource Technologies (SRTs) impose precise constraints on membrane selection. Conventional membranes can be broadly categorized as either polymers or ceramics. Commercially available polymer membranes suffer from issues such as low selectivity, limited mechanical robustness, and susceptibility to swelling in aqueous environments (Linkov and Belyakov, 2001; He et al., 2015; Hong et al., 2015). To address these challenges, the adoption of ceramic membranes in SRT has garnered increasing attention. Ceramic membranes, such as sodium beta-alumina, exhibit excellent mechanical stability and high selectivity but demonstrate limited chemical stability in seawater (Kim et al., 2016b). Notably, NASICON (Sodium Super Ionic Conductor), another ceramic material that conducts Na+, has been reported to remain stable in seawater while showcasing high Na+ selectivity and conductivity (Kim et al., 2016b; Song et al., 2016).
NASICON was initially discovered by Goodenough et al. (1976), and a digital image of the NASICON solid electrolyte is presented in Figure 3C. The representative composition of NASICON,Na1+xZr2SixP3-xO12 (0 ≤ x ≤ 3), features a corner-sharing structure of tetrahedral SiO4, PO4, and octahedral ZrO6 (Figure 3A) (Hwang et al., 2019). Various research efforts have explored the application of NASICON in battery technology, including Na-S batteries, seawater batteries, and sodium solid-state batteries (Kim et al., 2016b; Lu et al., 2021; Shen et al., 2021; Kim et al., 2022c). Additionally, NASICON has found use as a component in gas sensors. Sadaoka (2007) developed a CO2 sensor with the structure Pt/Li2CO3/NASICON/Pt, effectively addressing issues of low reproducibility and long-term stability seen in conventional CO2 sensors. Furthermore, NASICON can function as a CEM due to its Na+-conducting properties. The primary focus of CEM research has centered on selectivity, as high selectivity not only improves the purity of desired substances in the product but also reduces the energy loss required for extracting the target substance. In comparison to polymeric ion exchange membranes, which often exhibit low ion selectivity and unwanted water migration, Kim et al. (2022b) observed superior performance in NASICON-equipped electrodialysis for extracting pure NaCl from seawater.
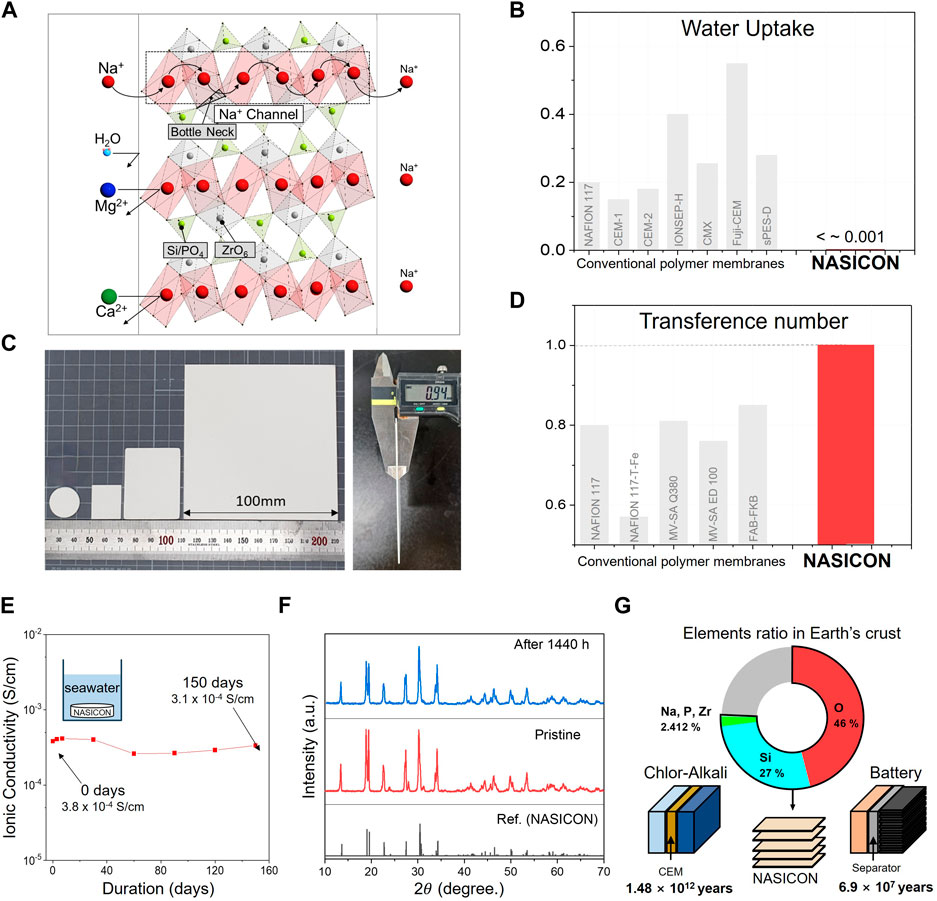
FIGURE 3. (A) Schematic representation of NASICON structure and ion transporting mechanism. (B) Comparison of water uptake between NASICON and polymeric cation exchange membranes (CEMs). (C) Digital images showcasing various sizes of NASICON. (D) Comparison of transference numbers between NASICON and various polymeric CEMs and cation-anion exchange membrane pairs. (E) Changes in ionic conductivity of NASICON with respect to seawater immersion time. (F) Comparison of XRD peaks between NASICON and seawater-immersed NASICON. (G) Elements ratio comprising NASICON in Earth’s crust and calculated years of NASICON availability in the CA field (left), and calculated years of NASICON availability in the battery field (right).
To fully leverage NASICON’s versatility in resource production from seawater, several critical factors must be considered: 1) ionic conductivity, 2) mechanical strength, 3) water uptake, 4) ion stability, and 5) chemical stability. Each of these factors is comprehensively examined and discussed in relation to NASICON’s properties as a membrane.
NASICON exhibits ionic conductivity ranging from 10−4 to 10−3 S·cm−1 at room temperature, a competitive range with that of organic solid electrolytes (10−6–10−3 S·cm−1) (Zhao et al., 2018). The ion-conducting mechanism in NASICON is determined by the Na+ sites (Na1, Na2, and Na3) and bottlenecks through which the ions migrate. Four sites exist in the NASICON formula unit, two-thirds of which are occupied (Rao et al., 2021). Na+ existing in the interstitial site must pass through a triangular bottleneck formed by three oxygen atoms, which has a cross-sectional area of 5.223 Å2 (Lu et al., 2018). Increasing the bottleneck size can reduce the activation energy for Na+ conduction, consequently affecting the ionic conductivity of NASICON. Ma et al. (2016) introduced scandium doping in NASICON, increasing the bottleneck size to 5.4 Å2, resulting in an ionic conductivity of 4.0 × 10−3 S·cm−1. Strategies to enhance the ionic conductivity of NASICON have been proposed, including aliovalent doping and modifications to the sintering process. For instance, Oh et al. (2019) added Na2SiO3 as a sintering aid and reduced the sintering temperature, achieving significantly higher ionic conductivity (1.45 × 10−3 S·cm−1) compared to bare NASICON (0.36 × 10−3 S·cm−1).
Mechanical strength is a crucial consideration in the manufacturing and assembly of systems utilizing NASICON. The fracture strength of Na3Zr2Si2PO12 was found to be 73 MPa, relatively lower compared to that of Na-beta-alumina (200 MPa) (Fertig et al., 2022; Wolfenstine et al., 2023). One contributing factor to the lower fracture strength of Na3Zr2Si2PO12 is the presence of ZrO2 as a secondary phase. Go et al. demonstrated that ZrO2-deficient NASICON (Na3.1Zr1.55Si2.3P0.7O11) exhibited improved mechanical strength (95 MPa) (Go et al., 2021).
Water uptake, indicating the amount of water absorbed by the membrane, is a crucial parameter affecting the water selectivity of an ion-exchange membrane. High water uptake in polymers leads to high hydrophilicity, and consequently, high water transport. This parameter can be calculated as
where mw and md denote the masses of the wet and dry membranes, respectively (Izquierdo-Gil et al., 2012). Qiu et al. (2021) demonstrated that water transport occurs in ion-exchange membranes with water uptake. When comparing the water uptake of polymeric CEMs and NASICON in labscale and commercial-scale applications (Figure 3B), it is evident that polymeric ion exchange membranes typically have a range of 0.1–0.6, whereas NASICON exhibits a water uptake close to zero, indicating lower water transport and higher water selectivity (Balagopal et al., 1999; Izquierdo-Gil et al., 2012; Avci et al., 2020).
The transfer number determines the ion selectivity of a membrane. The highest attainable value for the transference number was 1, indicating that the entire current was utilized to move a specific ion. Therefore, a higher transference number in the membrane is highly desirable to enhance the efficiency. Figure 3D compares the sodium ion transference numbers of the CEM, cation-anion exchange membrane pairs, and NASICON (Kameche et al., 2007; Kang et al., 2018; De Schepper et al., 2019). The transference number of polymer membranes ranges from 0.5 to 0.8, whereas that of NASICON is reported to be close to one (0.99999) (Song et al., 2016). Kim et al. demonstrated that a NASICON membrane exhibited high Na+ ion selectivity by effectively blocking Ca2+ and Mg2+ ions dissolved in seawater (Kim et al., 2022b).
For NASICON to be employed in SRTs, its stability in seawater must be established. Figure 3E depicts the change in the ionic conductivity of NASICON over time immersed in seawater (Hwang et al., 2019). In contrast to sodium beta alumina, known for its Na+ ion conductivity but susceptibility to moisture (Kim et al., 2016b), NASICON exhibited almost no change in ionic conductivity after 150 d, from 3.8 × 10−4 to 3.1 × 10−4 S·cm−1. Additionally, no significant change in the phase structure was observed in the XRD peaks between the bare sample and the sample immersed in seawater for 1,440 h (Figure 3F). In the extended experiment, NASICON demonstrated robust chemical compatibility with seawater, preserving its electrochemical stability for over a year (Wi et al., 2020).
Alongside the chemical stability of NASICON in seawater, it is important to consider its susceptibility to microorganisms present in seawater. This could potentially diminish the active surface area of NASICON, thereby reducing the overall performance of NASICON-based SRTs. Various established mitigation technologies, such as continuous UV irradiation or chemical treatments, can be implemented to mitigate bio-fouling on the surface of NASICON (Matin et al., 2011).
Along with these advantages, the elements composing NASICON are abundant on Earth. It is estimated that the elements in the Earth’s crust constituting NASICON make up 75.412% (WolframAlpha, 2014). Figure 3G shows the projected usable years when NASICON is applied to conventional electrochemical technologies that utilize ion exchange membranes (CA) and batteries. When CEM is replaced with NASICON in CA systems, it is estimated to be usable for approximately 1.48 × 1012 years, based on the elemental content in the Earth’s crust (O'Brien et al., 2005; WolframAlpha, 2014; The Essential Chemical Industry, 2018). Similarly, assuming that NASICON would replace the polymer separator in batteries, it was calculated that NASICON would be available for 690 billion years (WolframAlpha, 2014; Youme, 2022).
3.2 Energy storage with Na+ in seawater
Na+ in seawater hold promise as an energy carrier, akin to the roles played by Li+ in lithium-ion batteries and Pb2+ in lead-acid batteries. This potential is exemplified by the rechargeable seawater battery (SWB), which relies on NASICON as a crucial component for selective Na+ transfer from seawater (Kim et al., 2014a; Kim et al., 2014b). Unlike many conventional batteries ill-suited for water, especially saltwater environments, SWBs exhibit robust compatibility. As illustrated in Figure 4A1, the battery structure comprises a negative electrode, a solid electrolyte (NASICON), and a positive electrode (seawater) (Hwang et al., 2019). The solid electrolyte comes into direct contact with seawater, facilitating Na+ transport between the electrodes. The negative electrode consists of Na metal as the active material and is immersed in a non-aqueous electrolyte, acting as a buffer layer to facilitate Na+ transfer. Similar to other aqueous or underwater-operating batteries, this battery benefits from controlled heat and explosion prevention in its operation under seawater conditions (Chao et al., 2020; Chen et al., 2020).
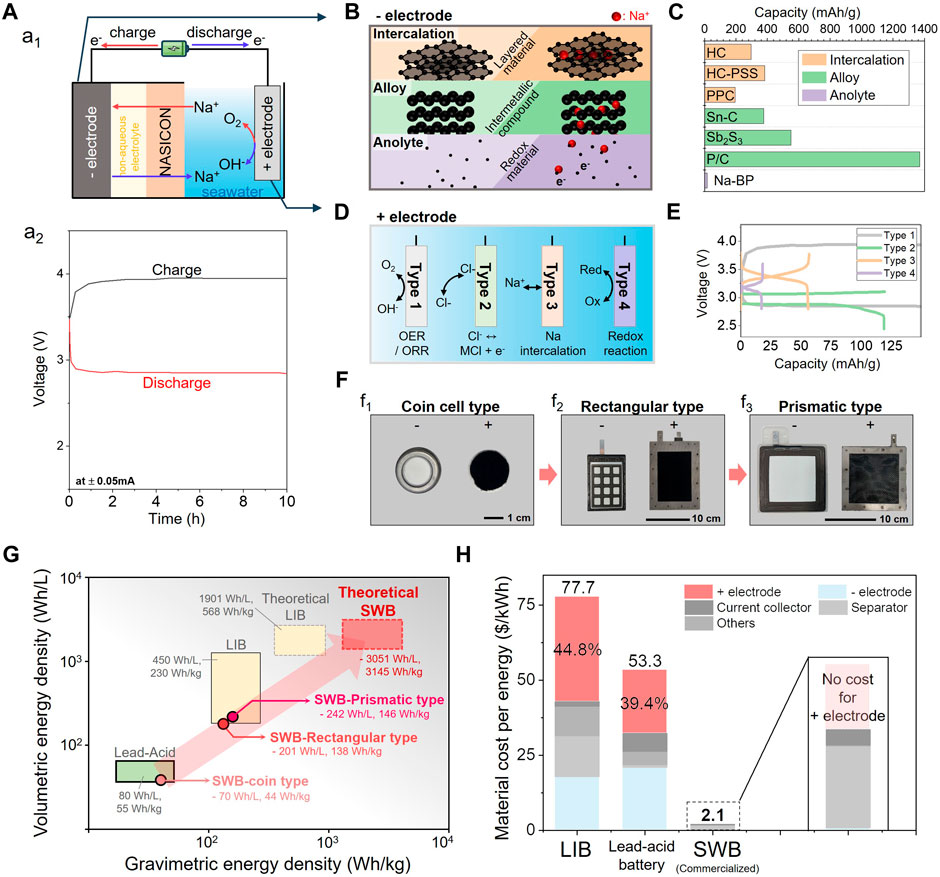
FIGURE 4. (A) (A1) Schematic of NASICON-based energy storage system (seawater battery) and (A2) typical charge-discharge voltages profile of seawater battery operated at a current of 0.05 mA. (B) Schematic illustrations presenting the mechanisms of three negative active material types for SWBs. (C) Specific capacities of various negative electrodes categorized into three types. (D) Schematic illustrations of four types of reactions for positive electrode materials of SWBs. (E) Charge-discharge voltage profile for three types of negative electrode. (F) SWBs unit cell development chronicle from (F1) coin cell type, (F2) rectangular type, to (F3) prismatic type. (G) The volumetric and gravimetric energy densities of various SWB types and other batteries. (H) Material cost per energy and cost breakdown for lithium-ion battery, lead-acid battery, and seawater battery.
SWBs operate based on redox reactions involving Na+ (Eq. 1) at the negative electrode and seawater at the positive electrode. Ambient oxygen gases are utilized at the positive electrode for discharging electricity, involving a reduction reaction known as the oxygen reduction reaction (ORR) (Eq. 3). Notably, the thermodynamically favored oxygen evolution reaction (OER) predominates over chlorine evolution (Eq. 6) or the formation of chlorine oxyacids (Eqs 4, 5) during charge cycling. The overall reactions are expressed as (Eq. 8):
Figure 4A2 displays the typical charge-discharge voltage profile of an SWB. While the SWB boasts a theoretically high cell voltage of 3.48 V, considerations of the overpotential, particularly due to the four-electron transfer OER/ORR pathway, may result in substantial polarization, indicating a gap between the charge and discharge voltages (Hwang et al., 2019).
Following the conceptual proof of the SWB in 2014 (Kim et al., 2014b), extensive research and development efforts have been dedicated to component/material selection and cell platform design. The progress in the development of this system can be categorized into three main areas: 1) negative electrodes, 2) positive electrodes, and 3) cell platform design. The upcoming paragraphs delves into detailed accounts of research advancements by drawing on published studies.
The negative electrode plays a crucial role in determining the capacity of an SWB. Na metal stands out as the most promising material due to its ability to offer a large specific capacity (1,166 mA·g−1) and enable the construction of “anode-free” SWBs for more efficient utilization of seawater as an active component (Kim et al., 2014a). However, Na is known to be more reactive compared to Li metal (Guo et al., 2017; Yang et al., 2017), introducing challenges related to the reversibility of deposition/stripping and the safety of cell operation (Zheng et al., 2019). Addressing these concerns necessitates the implementation of an effective strategy for forming a stable passivation layer (SEI) on the surface (Choi et al., 2018; Go et al., 2018), ensuring reversible behavior through the engineering of the anode components.
Owing to the challenges associated with the use of Na metal, various alternatives for negative electrodes have been explored. As illustrated in Figure 4B, these electrodes can be classified into three types: intercalation, alloying, and anolyte.
In the intercalation type (first row of Figure 4B), atoms or ions are reversibly integrated into layered materials to form interlayer compounds. Representative intercalation materials include carbon-based variants such as hard carbon (HC), hard carbon-poly(4-styrenesulfonate) composites (HC-PSS), and pine-pollen carbon (PPC) (Figure 4C). Among these, HC, with a theoretical capacity of 300 mA·g−1, is the most prevalent material used for battery electrodes (Park et al., 2016; Kim et al., 2018b). When employed as the negative electrode of an SWB, Park et al. demonstrated its feasibility and confirmed a discharge capacity of 296 mAh·g−1, aligning with its theoretical capacity (Park et al., 2016). Additional carbon-based options include enhanced HC-PSS composites and PPC derived from biomass. PSS [poly(4-styrenesulfonate)] exhibited a coulombic capacity of 146 mAh·g−1 and a discharge potential similar to that of HC. When combined with HC, it expands the specific capacity and coulombic efficiency of HC to 383.2 mAh·g−1 and 95.8%, respectively, at a current density of 0.125 mA·cm−2 (Lim et al., 2021). Additionally, research on PPC, an ecologically friendly and renewable biomass-derived material, revealed a capacity of 195 mAh·g−1 and 98% coulombic efficiency at a current density of 50 mA·g−1 (Kim et al., 2021a).
Alloy-type materials, shown in the second row of Figure 4B, offer a higher specific capacity, resulting in an increased energy density. Several studies have investigated the incorporation of alloy-type materials as the negative electrode for SWBs, such as tin-carbon nanocomposite (Sn-C), antimony sulphides (Sb2S3), and amorphous red phosphorous-carbon (P/C) composite, which form Na-rich intermetallic compounds through alloy reactions.
Among these materials, Sn-C stands out because it is based on Sn and has a high theoretical capacity. To address the significant volume changes that occur in tin during charging and discharging, a composite of Sn-C was created, in which tin nanoparticles were enclosed within a carbon matrix (Kim et al., 2014b). This enhancement resulted in the formation of Sn-C, which demonstrated an impressive specific capacity of 377 mAh·g−1 at 0.05 mA·cm−2. Hwang et al. employed a-Sb2S3 nanoelectrodes as the negative electrode, showing a remarkable specific capacity of 550 mAh·g−1 at 50 mA·g−1 (Hwang et al., 2016). Moreover, amorphous red phosphorus-carbon (P/C) has great potential because of its high reversible capacity, which contributes to its improved cyclability. Traditionally, P/C has seen limited adoption as a battery electrode because of its low reversibility in the initial cycles. However, in the context of SWB systems, where Na+ is continuously supplied from seawater, P/C has found a suitable application as a negative electrode by compensating for the initial Na+ consumption (Kim et al., 2018a). It exhibited a specific capacity of 1370 mAh·g−1 at a current density of 100 mA·g−1.
The anolyte, serving as a bifunctional liquid electrolyte, offers a compelling avenue for augmenting both battery capacity and reversibility. It possesses redox-active properties and dual ion and electron conductivities while simultaneously serving as a liquid electrode and electrolyte. NASICON enables the incorporation of the anolyte into the SWB without causing short circuits, even with the electron conductivity of the anolyte, as NASICON effectively blocks electron transfer between the negative electrode and seawater (Yang et al., 2021). Sodium biphenyl (Na-BP in DEGDME) is a representative anolyte (Figure 4C). Research indicates that Na-BP effectively mitigates issues such as gas evolution, Na dendrite growth, and dead capacity formation, which are common concerns with conventional electrodes and liquid electrolytes. It demonstrated a specific capacity of 17 mAh·g−1 at 0.25 mA·cm−2 (Kim et al., 2020b), offering a substantial advantage by supplementing the existing electrode capacity.
The positive electrode serves as the core of the SWB and provides a constant supply of water and Na+. Figure 4D displays four potential types of positive electrodes encompassing the foundational OER/ORR mechanism in seawater and ambient air: OER/ORR (Type 1), Cl capture (Type 2), Na intercalation (Type 3), and redox reactions (Type 4).
The OER/ORR mechanism is the cornerstone of the original concept of SWBs. This process relies on the abundant O2 present in seawater, providing substantial gravimetric and volumetric specific capacities (Figure 4E). However, reactions involving four electrons are kinetically less favorable than two-electron-based reactions, such as the formation of hypochlorite (ClO−) during charging (Eq. 4) or the ORR via a two-electron reduction pathway during discharge (Eq. 9). These two-electron reactions adversely affect the voltage efficiency of the batteries. Consequently, extensive research efforts, ranging from current collectors (Han et al., 2018; Zhang et al., 2018; Lee et al., 2020b; Park et al., 2020) to electrocatalysts (Abirami et al., 2016; Cheon et al., 2016; Shin et al., 2019; Lee et al., 2020a; Ryu et al., 2022), have been undertaken to enhance the sluggish kinetics of the OER and ORR processes, thereby reducing significant voltage discrepancies.
Alternatively, electrodes based on Cl capture/release have been explored to narrow the voltage gap. This approach employs a chloride ion-capturing electrode (CICE) similar to an Ag foil. The reversible Cl capture/release reaction is expressed as follows:
Kim et al. (2016a) reported that for the Ag electrode, the obtained voltage gap and voltage efficiency were 0.3 V and 90.3%, respectively, under a current density of 4.76 mA·g−1 (refer to Figure 4E). This represents an improved electrochemical performance compared with the OER/ORR approach. However, the high cost of Ag foil poses a significant obstacle to its commercial viability. Consequently, potential chloride storage materials such as Bi (Nam and Choi, 2017), BiOCl (Chen et al., 2017), polypyrrole (Kong et al., 2019), and polysilsesquioxane (Zhao et al., 2019) are being considered as cost-effective alternatives.
Positive electrodes utilizing Na+ intercalation/deintercalation can counteract the sluggish kinetics of OER/ORR processes. Most of the materials that intercalate Na+ into conventional sodium ion batteries are suitable for these electrodes. For example, Senthilkumar et al. (2017) employed nickel hexacyanoferrate (NiHCF) as the positive electrode material, according to Eq. 11:
It demonstrates small voltage gaps during cell operation owing to the rapid intercalation/deintercalation reaction and high reversibility, with seawater acting as an aqueous electrolyte (Figure 4E) at 20 mA·g−1 (Senthilkumar et al., 2017). Moreover, its affordability and straightforward manufacturing processes enhance its practicality and economic viability. However, the energy is constrained by the amount loaded during the electrode coating process, resulting in a lower capacity than that of open seawater electrodes.
Efforts have also been directed towards augmenting the output of rechargeable SWBs by employing redox reactions with NaHCF [Na4Fe(CN)6∙10 H2O], a solution of redox couples. The redox reaction of NaHCF is expressed as
As illustrated in Figure 4E, operating at a charge-discharge rate of 0.25 mA·cm−2, the one-electron redox reaction of NaHCF demonstrates superior kinetics compared to the four-electron OER/ORR process. Additionally, it exhibited exceptional charge-discharge performance with 100% Coulombic efficiency (Senthilkumar et al., 2018). In a closed container, a NaHCF solution can serve as a substitute for seawater in a conventional SWB system, even though Na+ is no longer continuously supplied from an open seawater system. However, because of its suitability for compact and high-power applications, ongoing research has focused on increasing the concentration of NaHCF to further enhance its energy density.
In addition to material studies on negative and positive electrodes, efforts have been made to enhance the practical energy density of SWBs by refining the cell structure design. The design and development of these SWB cells are directly related to the possibility of molding NASICON, a solid electrolyte. In other words, the design of SWB cells was developed using the NASICON molding ability, large area, high strength, and stability. Additionally, the system’s successful and secure operation relies heavily on ensuring the complete sealing of the negative electrode, which is equipped with the molded NASICON, to prevent any seawater from leaking into the electrode. As shown in Figure 4F, the SWB designs progressed from coin-to rectangular- and prismatic-type cells (Kim et al., 2022c). Establishing a standardized cell structure is crucial for demonstrating its feasibility. In addition, consistent laboratory-scale experiments should be conducted using well-established platforms to facilitate extensive material studies. For this purpose, coin- and flow-type cell testers were developed to comprehensively assess the electrochemical performance of the SWB (Han et al., 2018). The battery comprises a negative electrode incorporating NASICON (left in Figure 4F1) and a current collector (carbon cloth) as the positive electrode (right in Figure 4F1). Unlike other lithium-ion battery systems, the SWB cell structure has a structure with separate positive and negative parts, making it advantageous for maintenance of NASICON. When a problem occurs with the NASICON plate, repairs can be made by replacing only the negative electrode (Kim et al., 2022c). The standardized coin cell provided a testbed for system component development prior to large-scale experiments.
Subsequently, a rectangular type was introduced to enhance battery capacity and electrochemical performance (e.g., current and power). A negative electrode with NASICON (left in Figure 4F2) was constructed by arranging 24 square-type NASICONs (12 per side), and a carbon cloth with a titanium mesh enclosed the negative electrode through spot welding (Kim et al., 2021b). This pilot automated battery-manufacturing process enables the production of cell stacks or racks (Kim et al., 2022a). Further efforts were made to minimize unnecessary dead space in the rectangular type owing to the patchwork of NASICONs requiring sealing, which is electrochemically inactive (Kim et al., 2022c). This led to the development of a prismatic type utilizing a rigid frame and a single large NASICON with an extended space (Figure 4F3). This advancement improves the voltage efficiency through a uniform current distribution and allows more active materials to be stored, resulting in an increased energy density.
SWB development is ongoing to achieve the theoretical energy density targets (3,051 Wh·L−1, 3,145 Wh·kg−1), surpassing the theoretical values for lithium-ion batteries (Figure 4G). The volumetric and gravimetric energy densities of SWB have progressed in stages from 70 Wh·L−1 and 44 Wh·kg−1 (coin type), comparable to lead-acid batteries, to 242 Wh·L−1 and 146 Wh·kg−1 (prismatic type), respectively, and competitive with lithium-ion batteries (Kim et al., 2022c). All research and development activities, from component/material selection to cell design, are converging towards the mature stage of this technology, promising sustainable operation with a high energy density.
Upon full commercialization and attainment of the theoretical energy density, Son et al. (2021) projected the overall material cost per unit energy of SWB to be 2.1 US$ kWh−1 (Figure 4H). This is primarily attributed to the cost-saving use of open seawater as a positive electrode, as well as the utilization of inexpensive, abundantly available battery components. In comparison, lithium-ion batteries and lead-acid batteries have reported material costs of 77.7 US$ kWh−1 (Wentker et al., 2019) and 53.3 US$ kWh−1 (Matteson and Williams, 2015), respectively, with the positive electrode accounting for roughly 40% of the total cost in both cases. In this regard, the exclusion of positive electrode costs in the SWB system highlights its substantial advantages for various applications. From component/material selection to cell design, developments are converging towards the mature stage and offer an economically compelling path for the next-generation of batteries via the inexpensive mass productivity of NASICON.
3.3 Hydrogen storage with water of seawater
Hydrogen boasts the highest energy density per unit mass of fuel, standing at 142 MJ·kgH2−1 (Usman, 2022). However, its energy per unit volume is relatively low (0.01 MJ·LH2−1) due to its low ambient-temperature density, necessitating the development of advanced storage methods to achieve higher energy densities (Durbin and Malardier-Jugroot, 2013). Traditional physical hydrogen storage methods typically involve harsh conditions, such as high compression pressure (800 bar) or cryogenic temperatures (−250°C) (Schlapbach and Züttel, 2001).
On the other hand, alkali metals like Na offer an effective means of storing hydrogen under normal temperature and pressure conditions, as they generate hydrogen gas when reacting with water. In this context, NASICON can be employed for electrolysis to obtain Na metal directly from natural seawater (Eq. 1). This differs from the traditional process of harvesting Na metal, which requires the purification and dehydration of NaCl (Xi et al., 2020). In the traditional process, the dehydration of NaCl is crucial because water may cause a hydrogen evolution reaction rather than Na metal formation at the positive electrode. In contrast, NASICON allows Na metal harvesting from seawater without interference from water splitting. This is achieved by creating an electrochemical bridge between seawater (the Na+ source) and the electrode (Na metal), providing a pathway for Na+ while preventing water permeation to the electrode. The concept of a NASICON-based hydrogen storage system can be realized using an SWB platform.
As depicted in Figure 5A, this process involves the migration of Na+ from seawater to the negative electrode, resulting in the formation of Na metal during the charging process (Eq. 1). Previous studies have confirmed the selective extraction of Na metal from seawater, with electrodeposition occurring on the current collector at the negative electrode during charging process (Figures 5B, D, E). Simultaneously, various oxidation reactions, as discussed in the previous Section 3.2 (Figure 4E), such as the OER or Na deintercalation, can occur at the positive electrode. In the subsequent discharging process, the plated Na at the negative electrode undergoes oxidation and is released into water through NASICON. Meanwhile, electrons from the oxidized Na induce water electrolysis for hydrogen evolution at the positive electrode during discharge (Eq. 13).
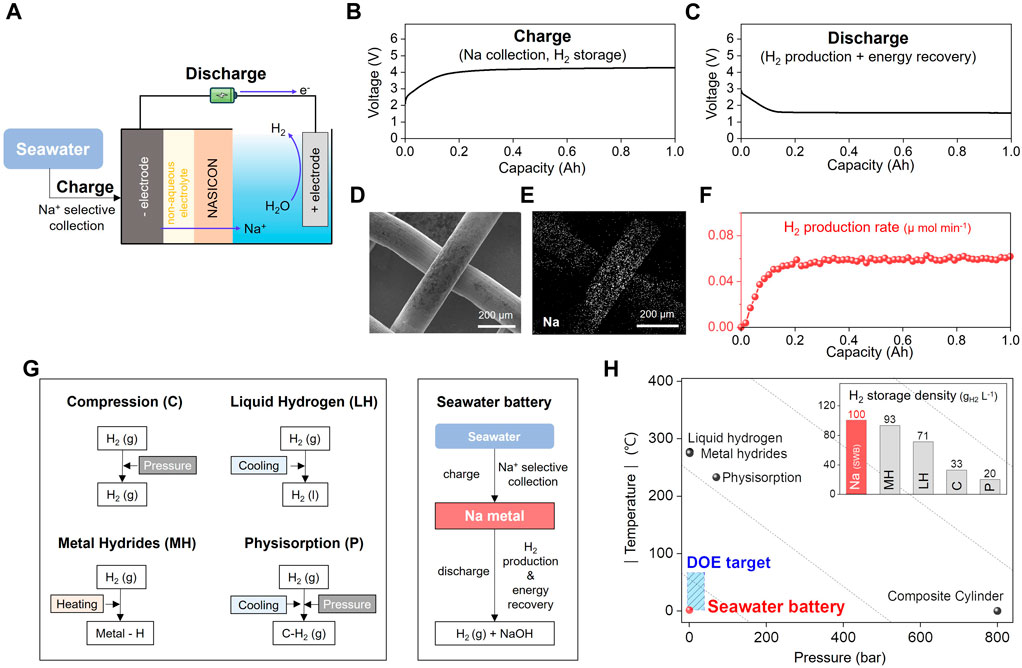
FIGURE 5. (A) Schematic of the seawater battery-based hydrogen storage system. Voltage profiles of seawater battery for hydrogen storage system during (B) charging process for Na collection and H2 storage and (C) discharging process for H2 production and energy recovery at 0.025 mA·cm−2. (D) SEM image of current collector in negative electrode after charging process and (E) corresponding EDS Na mapping image of current collector in negative electrode after charging process of current collector in negative electrode after charging process (F) Hydrogen production rate during discharging process at 0.025 mA·cm−2 (G) Schematic flowcharts of hydrogen storage process using compression, liquid hydrogen, metal hydrides, physisorption, and seawater battery. (H) Temperature and pressure required for hydrogen storage using liquid hydrogen, metal hydrides, compression, physisorption, and seawater battery.
Sharma et al. (2021) demonstrated the battery discharging process facilitated a steady hydrogen evolution rate of 0.05 μmol·min−1 (Figures 5C, F). This study suggests that Na metal plated on a negative electrode is an effective mediator for hydrogen storage. Thermodynamically, the oxygen reduction reaction (OER; Eq. 3) is predominant over the hydrogen evolution reaction HER (Eq. 2) for the positive electrode. However, they controlled the charge efficiency (CE) of the HER by employing electrode materials favorable for the HER or by modifying the operating conditions. Sharma et al. increased the CE of HER from 38% to 99% by increasing the current density from 0.2 to 2.0 mA·cm−2. This trend is attributed to the rapid exhaustion of residual oxygen, which is a reactant in the oxygen reduction reaction, at higher current densities. Impressively, the cyclic charging and discharging operations exhibited remarkable reversibility over 33 cycles spanning 400 h, with an average Faradaic efficiency of 95%; and the highest efficiency recorded was 100% during these 33 cycles.
A salient advantage of hydrogen storage via the SWB lies in its exceptional volumetric capacity, ranging from 42 to 218 g·L−1, under standard atmospheric pressure (1 atm) and ambient temperature (25°C) (Sharma et al., 2021). This outperforms the inherently low volumetric energy density of hydrogen, approximately 0.0824 kg·m−3, which translates to a scanty 0.01 MJ·L−1 H2 at ambient conditions and 8.5 MJ·L−1 H2 in its liquefied state (Durbin and Malardier-Jugroot, 2013; Preuster et al., 2017). In comparison, methane and gasoline boast volumetric energy densities of 0.04 MJ·L−1 H2 and 32 MJ·L−1 H2, respectively, surpassing hydrogen’s energy density by a considerable margin, posing a challenge to its commercial viability (Usman, 2022). As depicted in Figure 5G, various strategies have been proposed to enhance the volumetric energy density.
One of the most prominent hydrogen-storage methods involves the compression of composite cylinders (Durbin and Malardier-Jugroot, 2013). This technique enables the storage of hydrogen at high pressures (up to 800 bar) in cylindrical containers. This method is advantageous because it offers high rates of hydrogen filling and release (Zhang et al., 2016). Various metals such as metals, fiber resins, and metal/carbon fiber composites have been used as vessel materials (Durbin and Malardier-Jugroot, 2013; Barthélémy et al., 2017; Abdalla et al., 2018). This is because it effectively mitigates hydrogen diffusion and embrittlement. This approach yielded a volumetric capacity of 33 gH2·L−1 and an energy density of 5.7 MJ·L−1 (Rivard et al., 2019). However, the heavy weight and high cost of these materials pose challenges for this method (Usman, 2022).
Liquefying hydrogen at extremely low temperatures around −253°C can further enhance the volumetric capacity, achieving up to 71 gH2·L−1 due to the significantly higher density of liquid hydrogen compared to compressed hydrogen (Durbin and Malardier-Jugroot, 2013). However, the cost of liquefaction is so high that 30%–40% of net heating value is used for liquefaction. Hydrogen loss owing to evaporation, which is estimated at 1.5%–3% per day, is also a challenge for hydrogen liquefaction (Zhang et al., 2016). A vacuum vessel with double walls for rigid insulation is required to mitigate evaporation, which leads to an increase in weight and a reduction in energy density (Harris et al., 2004).
Additionally, the formation of metal hydrides, which can offer a volumetric capacity of up to 93 gH2·L−1, can be achieved through chemical interaction with metals or metal alloys upon heating 100°C (Schlapbach and Züttel, 2001). These metal hydrides can be formed through the direct reaction of hydrogen with the metal (Eq. 14) or through the electrochemical dissociation of water molecules (Eq. 15).
Metal hydrides offer higher storage capacity than compression or liquefaction at moderate temperatures and pressures. However, low adsorption and desorption kinetics, high temperatures for hydrogen release, and impurity gas formation during release pose challenges (Graetz, 2009; Ren et al., 2017).
Physisorption, which is a hydrogen storage method that relies on van der Waals interactions or dispersive forces, holds great promise. This is due to its low enthalpy of adsorption and desorption, typically ranging from 1 to 10 kJ·mol−1 (Tarasov et al., 2007), which facilitates efficient heat transfer management (Moradi and Groth, 2019). Additionally, physisorption eliminates the hydrogen loss resulting from byproduct formation. Materials with large surface areas, such as microporous carbon, metal-organic frameworks, and zeolites, have been extensively investigated for their suitability as adsorbents. Physisorption can store hydrogen within the range of 1–9 wt% at 77 K and at pressures ranging from 15 to 80 bar (Zhou et al., 2004; Farha et al., 2010; Lim et al., 2010; Langmi et al., 2014; Zhao et al., 2017). Notably, physisorption offers the distinct advantage of a high adsorption rate during hydrogen loading and unloading. However, it still presents challenges, particularly under harsh operating conditions, including cryogenic temperatures (0–120 K) and high pressures (15–80 bar).
Because conventional approaches are impeded by the substantial energy requirements for temperature and pressure control, hydrogen storage with low energy consumption is required for practical utilization. As illustrated in Figure 5H, the US Department of Energy (DoE) has outlined a target for hydrogen storage systems to attain a volumetric capacity surpassing 50 gH2·L−1 under pressures below 12 bar and temperatures ranging from −40°C–80°C (DoE, 2017). SWB achieves 218 gH2·L−1 under standard atmospheric pressure (1 atm) and ambient temperature (25°C), thus aligning with the target proposed by DoE. This is a notable advancement, as SWB presents a method for overcoming the typical irreversible nature of alkali-metal-mediated hydrogen storage. However, the relatively slow rate of hydrogenation and dehydrogenation, approximately 0.05 μmol·min−1, poses a challenge to the practical application of SWB in hydrogen storage (Sharma et al., 2021). This challenge is expected to be addressed by optimizing NASICON and advancing electrode materials to maximize the current density.
3.4 Sodium hydroxide production through seawater electrolysis
Sodium hydroxide, commonly known as caustic soda, serves a crucial role in various industries and households, including soap production, papermaking, neutralization, and bleaching (Kurt and Bittner, 2000). Traditionally, it is produced through an electrolytic process using pure saltwater (NaCl-aq) (Bommaraju et al., 2000). Natural seawater, primarily composed of NaCl, presents an attractive source for NaOH production. SWBs offer an effective means of selectively extracting Na+ from seawater to generate NaOH. At the core of this system lies the Na+-selective NASICON membrane, which facilitates the collection of Na+ from natural seawater. With NASICON, NaOH can be directly extracted from seawater without pretreatment while enabling energy storage and production. As depicted in Figure 6A1, during the charging process, Na+ in the seawater were collected at the SWB negative electrode, as described in Eq. 1. In the subsequent discharging process, NaOH is obtained by reacting Na+ from the negative electrode with the OH− generated at the positive electrode, as shown in Eqs 2, 3. The overall reaction formula is the same as that in Eq. 13.
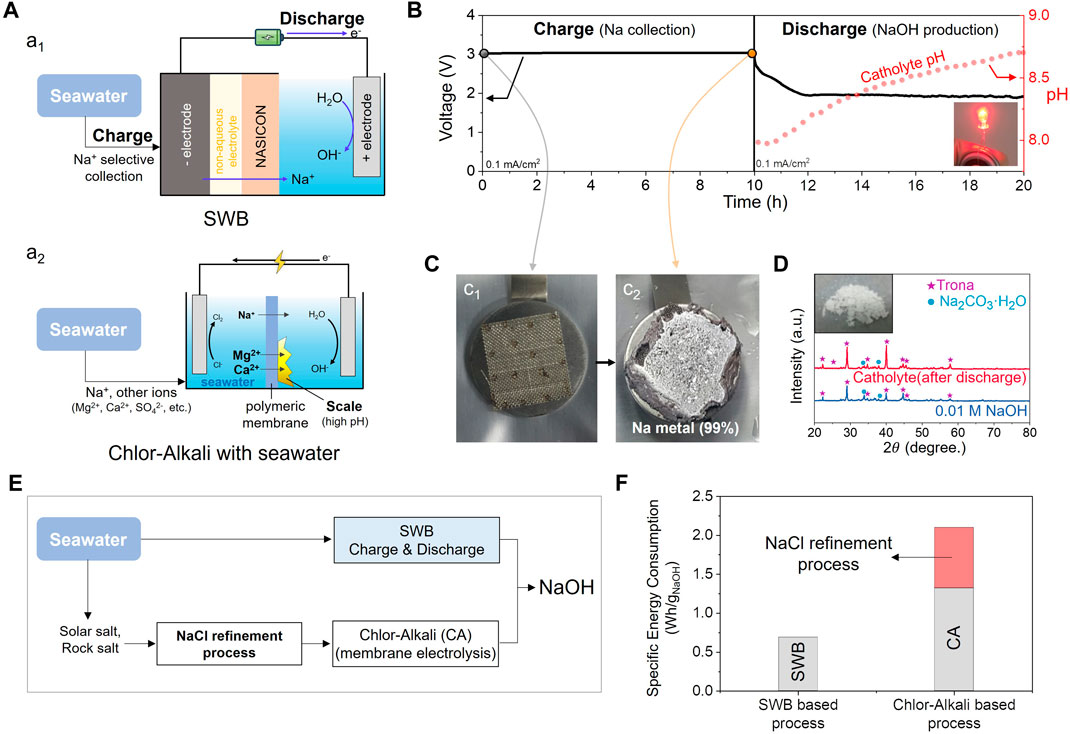
FIGURE 6. (A) Schematic illustrations of utilizing (A1, based on SWB system) and non-utilizing (A2. based on CA system) NASICON in NaOH production system from seawater. (B) Voltage profiles of the NaOH production system based SWB during the charging and discharging process with pH change at 0.1 mA·cm−2. (C) Digital images of Na metal deposited at the current collector in the negative electrode before and after the charging. (D) Comparison of XRD patterns of dried powder and 0.01 M NaOH solution after discharging-induced carbonation process. (E) Flow charts and (F) specific energy consumptions of SWB-based and CA system-based processes for NaOH production from seawater.
Bae et al. reported the production of NaOH from seawater using an SWB system, as illustrated in Figures 6B–D. In this demonstration, charging and discharging processes were conducted using a constant current mode (0.2 mA for 10 h within an SWB coin-type cell. The cell has a NASICON area of 2 cm2, corresponding to a current density of 0.1 mAcm−2. During the charging process, the sodium in seawater was collected in various forms in the negative electrode compartment of the SWB, including the formation of Na metal on the Ni mesh used as the anode current collector, as depicted in Figure 6C. As discussed in Section 3.2, alternative methods such as intercalation or alloying can also be employed for sodium collection.
During the subsequent discharging process, the stored energy is recovered, and the pH of the catholyte increases from 8 to 8.7 accordingly (Figure 6B). Additionally, this study demonstrated the formation of NaOH products through carbonation (Eq. 16). This process involves a reaction with atmospheric CO2, resulting in the formation of a solid precipitate known as Na2CO3. After the discharging process, the product catholyte was intentionally treated by carbonation, and a NaOH solution was treated following the same process as a reference. The overall carbonation process was conducted for several days at 35°C in ambient air to expedite the reaction. The XRD results provide evidence of the production of NaOH, as the major peaks of both samples coincide (Figure 6D). Furthermore, approximately 66% of the energy consumed during the charging process could be recovered during the discharging process.
An alternative method is the conventional electrolytic process, which is often referred to as the chlor-alkali (CA) process (Figure 6A2). However, this approach encounters challenges due to the presence of various ions and their byproducts in seawater, which trigger scale precipitation issues (Liu et al., 2020; Qiu et al., 2022; Yu et al., 2022; Yang et al., 2023). In the CA process, polymeric membranes, such as NAFION, are employed between the two electrodes. Unfortunately, these membranes permit the passage of ions such as Mg2+ or Ca2+. As these undesired ions migrate to the catholyte side, they directly react with the generated OH−, resulting in the precipitation of scale substances on the membrane surface, such as Mg(OH)2 and Ca(OH)2 (Eqs 17, 18). Moreover, these precipitates tend to accumulate near the electrodes, leading to severe damage to the entire system and impeding its long-term operation (Liu et al., 2022). Consequently, various sea salt refinement processes are required to meet the purity requirements for sustaining the chlor-alkali process (Figure 6E) (Du et al., 2018; Bae et al., 2019).
To evaluate the economic feasibility of NaOH production systems using the SWB and CA methods, a comprehensive analysis of both systems was conducted, considering energy and cost aspects (Figure 6E). The low ion selectivity of the NAFION membrane necessitates an elaborate purification process for salts extracted from seawater or salt lakes using conventional methods based on membrane electrolysis. This process aims to achieve a high-purity NaCl content and involves several intricate hybrid steps such as nanofiltration, electrodialysis, evaporation, mechanical vapor compression, chemical softening, and ion exchange.
Numerous studies have focused on reducing additional energy and costs associated with these processes. First, research has focused on nanofiltration (NF), a low-pressure membrane process known for its high divalent-ion removal rate (Zhou et al., 2015). However, as NF membrane performance improves, the challenge of managing the rising costs of these membranes must be addressed. Additionally, improvements have been made in electrodialysis (ED) systems, which generate brine containing high concentrations of Na+ and Cl− (Reig et al., 2014). However, despite its high NaCl concentration, concentrated brines fall short in terms of purity, requiring further refinement to meet CA process standards. Research has also explored the evaporation of interfacial seawater using solar power, demonstrating high evaporation efficiency but facing challenges related to ion concentrations (Zhang et al., 2021).
In contrast, the SWB system significantly reduced costs by directly utilizing seawater without the need for extensive pretreatment, as depicted in Figure 6F. Moreover, a substantial portion of the energy consumed during the charging process in the SWB system can be recovered during discharging, leading to remarkable energy efficiency. When comparing the two systems, the SWB system achieves a specific energy consumption of approximately 0.69 Wh·g−1 (Bae et al., 2019) per unit of production, marking a substantial reduction compared to CA’s 2.1 Wh·g−1 (Kumar et al., 2021). This represents a reduction of approximately 33% compared with the CA system, even when excluding the facility installation costs associated with maximum refinement.
Studies to date have confirmed the potential for energy- and cost-efficient NaOH production through proof-of-concept processes. However, in practical CA processes, a considerably high current density ranging from 4 to a maximum of 20 kA/m2 is applied, enabling rapid production rates (Hine et al., 2005; Mendoza et al., 2017). In contrast, the current NaOH production system utilizing NASICON has demonstrated efficacy at 1 A/m2. Therefore, by making progress towards increasing productivity through gradual scale-up, it is anticipated that this technology will evolve into a practical and valuable solution for various industrial applications.
3.5 Chlorine production through seawater electrolysis
Chlorine, renowned for its high reactivity, plays a pivotal role in industry as an oxidizing agent, bleaching agent, and disinfectant (Fair et al., 1948). It is a fundamental raw material in plastic production (Esselen and Bacon, 1938). Its primary production method involves the electrolysis of sodium chloride brine, similar to the process for sodium hydroxide (Lakshmanan and Murugesan, 2014). Chloride, comprising 55% of dissolved ions in seawater, is a plentiful resource. Nevertheless, as detailed in Section 3.4, the chlor-alkali technology for extracting target materials from seawater necessitates pretreatment due to the presence of various undesired ions (Casas et al., 2012).
In contrast, NASICON-based systems offer the direct utilization of seawater without the need for pretreatment. In this context, we delve into NASICON-based electrochemical systems, as depicted in Figures 7A1–A3. Figures 7A1, A2 elucidate the structure and mechanism of the Disinfection-Dichlorination battery (DD-battery) during the charging and discharging processes, respectively (Park et al., 2021). Additionally, Figure 7A3 presents an advanced NASICON-based electrolysis (N-Electrolysis) system utilizing a ceramic membrane between two electrodes, providing a competitive alternative to a simple electrolysis system with two electrodes (Figure 7A4) (Park et al., 2021). These systems rely on anodic chloride oxidation reactions at the positive electrode, resulting in total chlorine (TC) formation, with reaction potentials highly dependent on pH, as illustrated in Eq. 19.
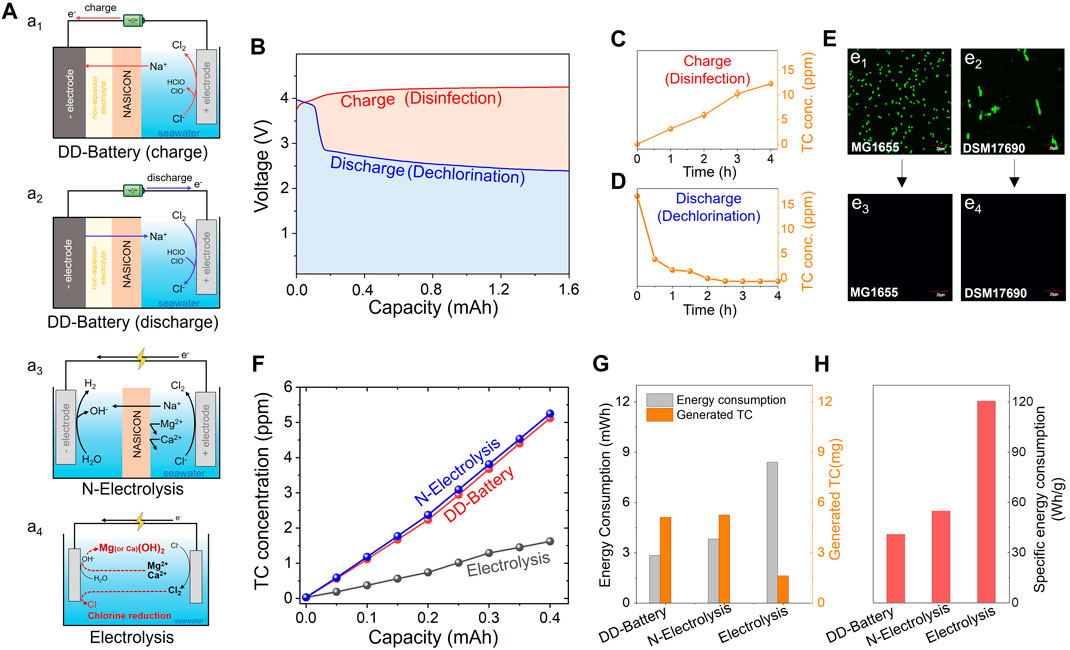
FIGURE 7. (A) Schematic illustrations of Disinfection and Dechlorination-battery (DD-battery, A1: charging, A2: discharging), N-electrolysis (A3), and electrolysis (A4) for chlorine production using seawater. (B) Voltage profiles of the DD-battery during charging (red solid line) and discharging (blue solid line) process at 0.2 mA. TC concentration changes during (C) charging and (D) discharging process. (E) Confocal microscopy images of MG1655 and DSM17690 before (E1 and E2 for MG1655 and DSM17690, respectively) and after charging (E3 and E4 for MG1655 and DSM17690, respectively). (F) TC concentration changes at 0.2 mA constant current in three systems: DD-battery (blue circles with solid line), N-electrolysis (red circles with solid line) and electrolysis (black circles with solid line). (G) Energy consumption and generated mass of TC in each system. (H) Specific energy consumption of each system.
The DD battery concept was introduced to produce TC at the positive electrode during charging for use as a disinfectant (Figure 7A1) (Park et al., 2021). Simultaneously, energy was stored by attracting and reducing Na+ at the negative electrode. The battery exhibited a subsequent discharging process to convert TC back into chloride (dichlorination) electrochemically, thereby mitigating the disinfectant’s toxicity, while recovering a significant portion of the energy used for TC production (Figure 7A2). Demonstrated the cyclic operation of a DD battery (at 0.2 mA current) with a coin-type cell. As depicted in Figure 7B, although 6.76 mWh of energy was consumed during the charging process, 3.92 mWh was recovered during the discharging process. Consequently, approximately 58% of the charging energy can be reused, with only 2.84 mWh consumed for producing disinfecting agents. Throughout the charging process, the TC concentration continuously increased to 12 ppm (Figure 7C). This study demonstrated that the bacteria MG1655 and DSM17690, characterized by confocal microscopy images at 530 nm, were completely disinfected after the charging process (Figure 7E). In the subsequent discharging process, the battery successfully removed the residual TC species by reducing them into Cl− (Figure 7D).
Furthermore, N electrolysis was proposed by simply converting the negative electrode of the DD-battery system to a NASICON membrane (Figure 7A3). The system employs NASICON as a membrane to facilitate Na+ conduction from the positive to the negative electrode, while blocking other materials such as Mg2+ or Ca2+ in seawater or oxyacids of chlorine (i.e., OCl−, HOCl, etc.) generated after seawater electrolysis. The study found two advantages of using NASICON for chlorine production compared to the conventional electrolysis system without a membrane (Figure 7A4). First, it allows for direct seawater utilization by effectively blocking the penetration of ions other than Na+, thereby avoiding issues related to scale formation and electrode/component deterioration associated with traditional methods. Secondly, it mitigates some of the reduction of Cl2, preventing the transformation of chlorine generated in the oxidation section back into Cl−.
This study collectively compared three systems (DD-battery, N-electrolysis, and electrolysis) in terms of production and energy consumption (Figures 7F–H). As depicted in Figure 7G, the DD-battery (5.12 ppm) and NASICON-based electrolysis (5.25 ppm) systems produced approximately three times more TC than electrolysis (1.62 ppm) under the same operating conditions (0.2 mA constant current). This disparity arises from the presence of NASICON, which effectively prevents the reduction of chlorine to chloride during electrolysis (Figure 7F). Furthermore, the DD-battery system enables energy recovery during the discharging process, resulting in lower energy consumption than that of N-electrolysis. In conclusion, the specific energy consumption of DD-battery, N-electrolysis, and electrolysis were 40.7, 54.7, and 120.5 Wh·g−1, respectively (Figure 7H). Overall, these findings suggest that NASICON offers two advantages for chlorine production: 1) direct seawater electrolysis and 2) low specific energy consumption for chlorine (or oxyacids of chlorine) production.
The introduced systems can also be compared in terms of safety. TC remaining in water has the potential to pose safety risks due to the generation of undesirable by-products and its high reactivity. Trihalomethanes (THMs), a representative by-product formed during the disinfection process, are of particular concern due to their potential carcinogenicity, which poses a severe health risk (Andersson et al., 2019; Dock et al., 2019). Additionally, the adverse effects of free chlorine that remains in disinfected seawater result from its reaction with organic compounds in seawater, which can have wide-ranging impacts on aquatic life and the ecosystem (Pan et al., 2019). As a practical approach, the electrolysis system is often operated in combination with an additional dechlorination system to remove TC from the water following disinfection treatment. This dechlorination system typically employs the dechlorinating agent sodium thiosulfate (Na2S2O3) to convert chlorine-based residues into harmless Cl−. Consequently, additional processes are necessary to address safety concerns, and this applies to the N-Electrolysis system as well. In contrast, the DD-battery system avoids the aforementioned safety issues. TC is effectively reduced back to Cl− during the discharge process, eliminating the risk associated with residual chlorine. Consequently, the DD-battery system offers a significant safety advantage over other systems.
Although NASICON enables energy-efficient direct seawater-based chlorine manufacturing systems, further advancements are required. Specifically, improvements should target the coulombic efficiency of the chlorine evolution reaction (CER) in seawater. Typically, two primary oxidation reactions occur competitively at the positive electrode: CER and oxygen evolution reaction (OER) (Adiga et al., 2022). Similarly, a high OER rate may interfere with chlorine production. When the desired reaction is CER, it is typically enhanced by maintaining optimal operating conditions with a pH range of 1–2 and a temperature of around 90°C, as practiced in the CA processes (Sugiyama et al., 2003). However, NASICON is fragile under such acidic conditions, limiting its applicability under favorable CER conditions (Karlsson and Cornell, 2016). Previous studies have reported that seawater conditions lead to lower Coulombic efficiencies for the CER compared with the OER (Khatun et al., 2021; Park et al., 2021). In this regard, various approaches can expand the applicability of NASICON-based chlorine production systems, such as the development of catalysts that promote the CER selectivity under seawater conditions or enhance the resistivity of NASICON under acidic conditions.
3.6 Seawater desalination and mineral production
Freshwater is an indispensable resource for various human activities, including agriculture, manufacturing, power generation, and household use (Bonamente et al., 2015). However, a significant portion of Earth’s water resources exists in frozen or saline forms, limiting direct accessibility. Seawater desalination, which produces 100 million cubic meters of freshwater daily, is a promising method for obtaining freshwater (Boretti and Rosa, 2019). Nevertheless, conventional desalination processes generate concentrated brine as a byproduct after separating freshwater from seawater. This brine poses environmental challenges when left untreated, disrupts marine ecosystems, and causes a sharp increase in salinity (Roberts et al., 2010).
In this context, NASICON plays a crucial role in separating seawater into freshwater and a pure NaCl solution, which holds significant value in the chemical industry without producing waste brine (Figure 8D). This separation process was implemented by constructing an electrodialysis (ED) system with NASICON, replacing the CEM in a traditional ED system. In addition to the NASICON-applied ED system (N-ED), we will explore a seawater battery for desalination (SWB-D) that is capable of simultaneous energy storage, seawater desalination, and NaCl production.
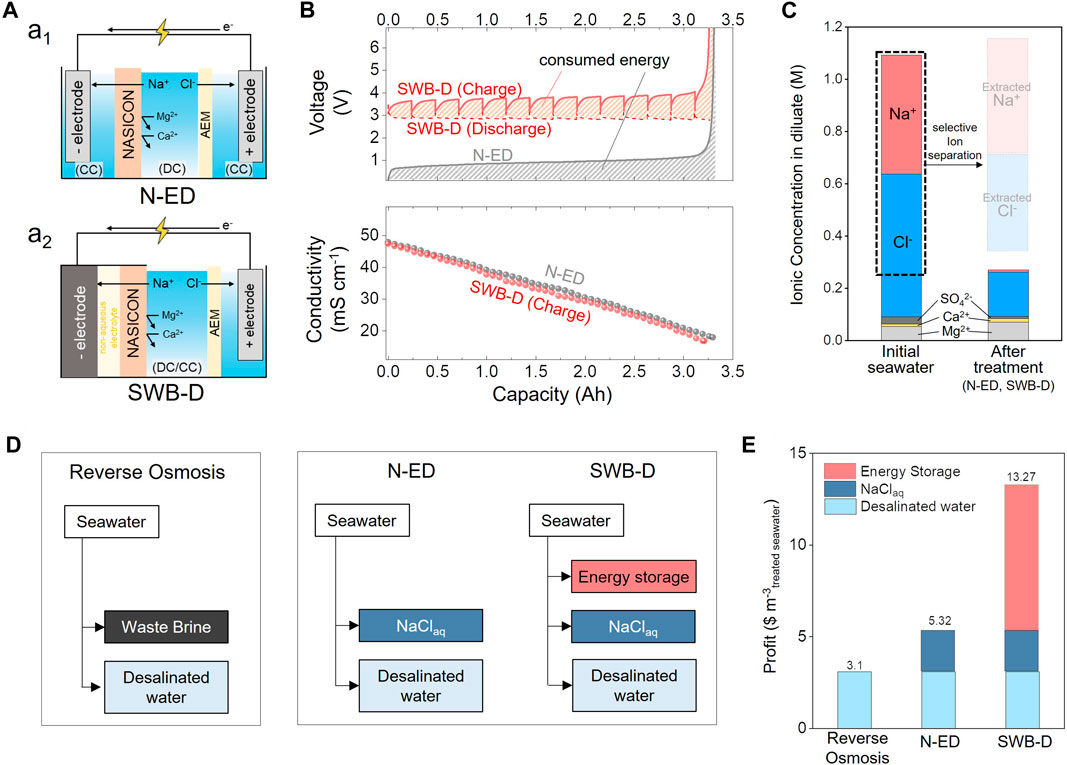
FIGURE 8. (A) Schematic illustrations of (A1) NASICON-based electrodialysis (N-ED), and (A2) Seawater battery-based desalination battery (SWB-D). (B) Voltage profiles of N-ED (black solid line) and SWB-D (red solid lines) during charging and discharging process at 2 Am−2 (C) Ion concentrations of seawater (before treatment, left) and desalinated water treated with N-ED, and SWB-D (right) (D) Flowchart diagrams of reverse osmosis, N-ED, and SWB-D. (E) Profits of desalination processes using RO, N-ED, and SWB-D.
As illustrated in Figure 8A1, the N-ED comprises a negative electrode, NASICON, an anion exchange membrane (AEM), and a positive electrode. The system is divided into two compartments: the diluate compartment (DC) between the NASICON and the AEM, where desalination occurs, and the concentration compartment (CC), where the ions are concentrated. By applying an electric field between the negative and positive electrodes, Na+ migrates from the DC to the negative electrode via the NASICON, whereas Cl− moves to the positive electrode through the AEM. This leads to a decreased salinity in the DC, resulting in desalinated water, whereas the CC becomes concentrated and produces brine. Kim et al. demonstrated the operation of an N-ED by replacing a polymeric CEM with a NASICON in the ED, thereby enabling seawater desalination in the DC and NaCl concentration in the CC. The conductivity of the DC decreased as the ionic concentration decreased (Figure 8B). Specifically, only Na+ and Cl− were selectively extracted from the DC and collected from the CC. According to the results of the ionic concentration change after the selective ion separation process, 22.2 kg of salt could be extracted from the CC by treating 1 m3 of seawater (Figure 8C). This highly pure NaCl product contributes significantly to profitability, with the price of pure NaCl rising to 0.1 USD per kg as purity approaches 100% (Sedivy, 2008). This translates into an additional profit of 2.22 USD, alongside a 3.1 USD profit from 1 m3 of freshwater (Panagopoulos, 2022) (Figure 8E).
As shown in Figure 8A2, SWB-D employs an AEM along with positive and negative electrodes (Kim et al., 2020a). It shares a similar structure to N-ED, utilizing the section between NASICON and the AEM as the diluate compartment (DC). During the battery charging process, Na+ in the DC migrates to the negative electrode via the NASICON, whereas Cl− moves to the positive electrode through the AEM, resulting in the desalination of seawater in the DC. Kim et al. observed a decrease in the conductivity of DC by charging SWB-D (Figure 8B). After desalination, desalted water was obtained from the DC. Subsequently, during the discharging process, the DC is transformed into a concentration compartment (CC). As the CC fills with water, Na+ from the negative electrode is transferred to the CC via NASICON, and Cl− flows to the CC via the AEM. As shown in Figure 8B, the energy used during charging process is partially recovered during discharging process. Notably, desalinating 1 m3 of seawater yields not only 22.2 kg of salt but also 44.16 kWh of recovered energy (Figure 8B). The energy-storage capability of SWB-D is vital because it directly contributes to economically viable seawater desalination. Energy storage is crucial for stabilizing renewable energy use, because it has the potential to minimize energy consumption costs and maximize profits through efficient energy distribution. Consequently, a profit of 7.95 USD (44.16 kWh x 18.02 ¢/kWh = 795 ¢) can be generated compared to N-ED (Figure 8E).
Various studies have propelled the use of SWB-D technology for more energy-effective and scalable battery desalination. Following a conceptual proof with a coin-type cell capable of treating 3.4 mL of seawater, Kim et al. (2022b) developed a rectangular cell platform capable of treating 250 mL of seawater at 20 mA. This development led to a reduction in specific energy consumption from 21.3 to 14.58 kWh·m−3. The study also revealed that SWB-D exhibited a lower energy consumption than N-ED or ED, primarily because of its energy-recovery process during discharging process. This novel system is distinct in that it allows successive desalination and salination via charging and discharging process, without requiring seawater exchange.
Various innovations have been explored to enhance the SWB-D. Son et al. (2022) introduced NiHCF as a positive electrode. This led to efficient Cl− diffusion due to surplus positive charge during charging process. Na+ intercalation in NiHCF during discharging process further facilitated Cl− diffusion. This innovation greatly increased energy efficiency, reaching 86% compared to the 61% for carbon. Park et al. (2022) replaced AEM with reverse osmosis (RO) membranes to improve ion diffusion. This modification resulted in a 36.8% reduction in charging time and a 40.5% decrease in salt removal compared with conventional SWB-D.
NASICON-based desalination systems offer promising economic and environmental benefits. Ligaray et al. (2020) studied the economic impact of SWB-D on seawater desalination and developed an innovative process model that integrates SWB-D and RO. The integrated system exhibited an energy consumption of 1.3 kWh·m−3, which was lower than that of RO.
Kim et al. (2022b) explored the role of selective Na+ transfer during desalination, enabling the production of pure NaCl, which is an invaluable resource in the food and chemical industries. This approach contrasts with conventional desalination processes, which generate brine waste containing various impurities. This breakthrough is significant for environmentally sustainable desalination because the disposal of concentrated brine waste from traditional seawater desalination poses environmental challenges (Mauguin and Corsin, 2005; Roberts et al., 2010). Extracting resources from concentrated brine not only reduces the brine salinity but also consumes salt. Industries such as the chlor-alkali industry, which consume 90 million tons of salt annually, require high-purity salts with minimal Mg2+ and Ca2+ concentrations (below 0.1 μg·L−1) (Sedivy, 2008). Although the production of high-purity salts from seawater remains challenging, NASICON-based desalination systems enable this through selective Na+ transfer via NASICON.
4 Discussion
This review delves into the intricate properties of NASICON ceramics while providing an extensive overview of NASICON-based electrochemical systems. These systems encompass a wide array of applications, including energy storage, hydrogen production, sodium hydroxide and chlorine synthesis, water treatment, and mineral extraction. The integration of NASICON in these domains marks a significant milestone in realizing the direct utilization of seawater. NASICON’s exceptional attributes—its remarkable Na+ selectivity, ionic conductivity, and seawater stability—position it as an exceedingly efficient membrane suitable for diverse Seawater Resource Technologies (SRTs), extending beyond the scope of this review. This presents an appealing solution for surmounting the challenges associated with SRT technology, characterized by stringent membrane requirements, and offers the potential to harness valuable resources from complex, multi-component seawater, constituting an abundant and sustainable supply.
Building on these distinctive NASICON features, we explored a spectrum of SRTs exhibiting remarkable potential across various research domains. NASICON forms the bedrock for the Seawater Battery (SWB), wherein Na+ acts as a carrier, facilitating the exchange between seawater and the negative electrode. Ongoing research on novel materials and cell designs promises to elevate SWBs into high-energy-density, cost-effective storage solutions that surpass conventional batteries. Similarly, NASICON’s membrane underpins a significant advancement in hydrogen storage technology, enabling the extraction of hydrogen from seawater using Na metal. This approach allows for high-density hydrogen storage under standard temperatures and pressures, which is a significant improvement over traditional methods requiring extreme conditions. In the NaOH industry, NASICON’s selective Na+ permeability provides a direct route for extracting NaOH from natural seawater, eliminating the need for pre-treatment. This streamlines the process while unlocking opportunities for energy storage and production. Moreover, NASICON-based electrochemical systems offer an efficient means to directly produce Cl2 from seawater, offering a promising alternative to conventional NaCl brine electrolysis, which requires pre-treatment. Lastly, in seawater desalination, NASICON-based systems differentially segregate fresh water from NaCl without generating concentrated waste brine. This addresses a fundamental challenge in conventional desalination processes where waste brine poses a significant threat to marine ecosystems. In summary, these applications leverage NASICON’s unique properties to enhance performance, showcasing practical instances where seawater proves to be a valuable resource across various industries.
While NASICON-based technologies hold immense potential, several challenges must be overcome before their practical application. Firstly, enhancing the performance of SRTs necessitates the development of high-performance membranes with improved ionic conductivity and physicochemical stability. Presently, the acceptable applied current density for NASICON-based electrochemical devices remains below ∼10 mA/cm2. This limit should be increased to extend its application to other technologies such as batteries (∼30 mA/cm2), hydrogen production from water electrolysis (1–3 A/cm2), or the chlor-alkali system (0.4–2 A/cm2). Areal resistance can be minimized by developing ceramic membranes thin enough to be comparable to polymeric membranes (∼0.1 mm), while still retaining sufficient fracture strength to withstand ceramic breakage. Comprehensive research on water-stable solid electrolytes, particularly NASICON and its derivatives, is imperative.
Secondly, the creation of a simple and large-scale production method for solid electrolytes is essential. The use of solid electrolytes as separators in electrochemical systems, for example, requires production and processing methods akin to those employed for existing polymer-based membranes, as opposed to the traditional ceramic production method. This shift is driven by the need for expediency, energy conservation, and the elimination of post-treatment processes. Additionally, scale-up should be further studied, factoring in the physical properties and processability of the solid electrolyte. The inherent rigidity of solid electrolytes limits the enlargement of membranes, necessitating research into scalable methods. Addressing these issues holds the potential to be a pivotal moment in the evolution of SRTs based on NASICON.
In conclusion, NASICON-based SRTs are poised to play a transformative role in global sustainability. In particular, those excel in separating impurities and enabling the selective movement of sodium ions. This advancement has propelled the extraction of valuable resources from seawater, an abundant source rich in assets, frequently hindered by impurities. With these possibilities in sight, NASICON-based technology is expected to usher in a new era, effectively addressing the escalating demands for energy, water, and industrial resources while addressing issues of inequality by securing new energy and industrial resources.
Author contributions
NK: Resources, Supervision, Visualization, Writing–original draft, Writing–review and editing. SK: Resources, Visualization, Writing–original draft. SJ: Resources, Visualization, Writing–original draft. HJ: Resources, Visualization, Writing–original draft. HL: Resources, Visualization, Writing–original draft. YK: Funding acquisition, Supervision, Writing–review and editing. W-GL: Funding acquisition, Supervision, Writing–original draft, Writing–review and editing. J-SP: Funding acquisition, Supervision, Writing–original draft, Writing–review and editing.
Funding
The author(s) declare financial support was received for the research, authorship, and/or publication of this article. This study was supported by the Korea Institute of Energy Technology Evaluation and Planning (KETEP) grant funded by the MOTIE (20215610100040, Development of 20 Wh seawater secondary battery unit cell) and the Circle Foundation (Republic of Korea) for 3 years since 2020 as Seawater Resource Technology Center was selected as the “2020 TCF Innovative Science Project” (grant number 2020 TCF Innovative Science Project–06).
Conflict of interest
Author J-SP was employed by 4TOONE Corporation.
The remaining authors declare that the research was conducted in the absence of any commercial or financial relationships that could be construed as a potential conflict of interest.
Publisher’s note
All claims expressed in this article are solely those of the authors and do not necessarily represent those of their affiliated organizations, or those of the publisher, the editors and the reviewers. Any product that may be evaluated in this article, or claim that may be made by its manufacturer, is not guaranteed or endorsed by the publisher.
References
Abdalla, A. M., Hossain, S., Nisfindy, O. B., Azad, A. T., Dawood, M., and Azad, A. K. (2018). Hydrogen production, storage, transportation and key challenges with applications: a review. Energy Convers. Manag. 165, 602–627. doi:10.1016/j.enconman.2018.03.088
Abirami, M., Hwang, S. M., Yang, J., Senthilkumar, S. T., Kim, J., Go, W.-S., et al. (2016). A metal–organic framework derived porous cobalt manganese oxide bifunctional electrocatalyst for hybrid Na–Air/Seawater batteries. ACS Appl. Mater. Interfaces 8 (48), 32778–32787. doi:10.1021/acsami.6b10082
Adiga, P., Nunn, W., Wong, C., Manjeshwar, A. K., Nair, S., Jalan, B., et al. (2022). Breaking OER and CER scaling relations via strain and its relaxation in RuO2 (101). Mater. Today Energy 28, 101087. doi:10.1016/j.mtener.2022.101087
Alsabbagh, A., Aljarrah, S., and Almahasneh, M. (2021). Lithium enrichment optimization from Dead Sea end brine by chemical precipitation technique. Miner. Eng. 170, 107038. doi:10.1016/j.mineng.2021.107038
Andersson, A., Harir, M., Gonsior, M., Hertkorn, N., Schmitt-Kopplin, P., Kylin, H., et al. (2019). Waterworks-specific composition of drinking water disinfection by-products. Environ. Sci. Water Res. Technol. 5 (5), 861–872. doi:10.1039/c9ew00034h
Avci, A. H., Rijnaarts, T., Fontananova, E., Di Profio, G., Vankelecom, I. F., De Vos, W. M., et al. (2020). Sulfonated polyethersulfone based cation exchange membranes for reverse electrodialysis under high salinity gradients. J. Membr. Sci. 595, 117585. doi:10.1016/j.memsci.2019.117585
Bae, H., Park, J.-S., Senthilkumar, S., Hwang, S. M., and Kim, Y. (2019). Hybrid seawater desalination-carbon capture using modified seawater battery system. J. Power Sources 410-411, 99–105. doi:10.1016/j.jpowsour.2018.11.009
Balagopal, S., Landro, T., Zecevic, S., Sutija, D., Elangovan, S., and Khandkar, A. (1999). Selective sodium removal from aqueous waste streams with NaSICON ceramics. Sep. Purif. Technol. 15 (3), 231–237. doi:10.1016/s1383-5866(98)00104-x
Bardi, U. (2010). Extracting minerals from seawater: an energy analysis. Sustainability 2 (4), 980–992. doi:10.3390/su2040980
Barthélémy, H., Weber, M., and Barbier, F. (2017). Hydrogen storage: recent improvements and industrial perspectives. Int. J. Hydrogen Energy 42 (11), 7254–7262. doi:10.1016/j.ijhydene.2016.03.178
Bommaraju, T. V., Lüke, B., O'Brien, T. F., and Blackburn, M. C. (2000). Chlorine. United States: Kirk-Othmer Encyclopedia of Chemical Technology.
Bonamente, E., Scrucca, F., Asdrubali, F., Cotana, F., and Presciutti, A. (2015). The water footprint of the wine industry: implementation of an assessment methodology and application to a case study. Sustainability 7 (9), 12190–12208. doi:10.3390/su70912190
Boretti, A., and Rosa, L. (2019). Reassessing the projections of the world water development report. NPJ Clean. Water 2 (1), 15. doi:10.1038/s41545-019-0039-9
Casas, S., Aladjem, C., Cortina, J., Larrotcha, E., and Cremades, L. (2012). Seawater reverse osmosis brines as a new salt source for the chlor-alkali industry: integration of NaCl concentration by electrodialysis. Solvent Extr. Ion Exch. 30 (4), 322–332. doi:10.1080/07366299.2012.686849
Casey, D. (2018). Constituents of seawater. Available at: https://salinity.oceansciences.org/learn-more.htm?id=54 (Accessed July 09, 2023).
Chao, D., Zhou, W., Xie, F., Ye, C., Li, H., Jaroniec, M., et al. (2020). Roadmap for advanced aqueous batteries: from design of materials to applications. Sci. Adv. 6 (21), eaba4098. doi:10.1126/sciadv.aba4098
Chen, F., Huang, Y., Guo, L., Sun, L., Wang, Y., and Yang, H. Y. (2017). Dual-ions electrochemical deionization: a desalination generator. Energy & Environ. Sci. 10 (10), 2081–2089. doi:10.1039/c7ee00855d
Chen, L., Cao, L., Ji, X., Hou, S., Li, Q., Chen, J., et al. (2020). Enabling safe aqueous lithium ion open batteries by suppressing oxygen reduction reaction. Nat. Commun. 11 (1), 2638. doi:10.1038/s41467-020-16460-w
Cheon, J. Y., Kim, K., Sa, Y. J., Sahgong, S. H., Hong, Y., Woo, J., et al. (2016). Graphitic nanoshell/mesoporous carbon nanohybrids as highly efficient and stable bifunctional oxygen electrocatalysts for rechargeable aqueous Na–air batteries. Adv. Energy Mater. 6 (7), 1501794. doi:10.1002/aenm.201501794
Delmas, C. (2018). Sodium and sodium-ion batteries: 50 years of research. Adv. Energy Mater. 8 (17), 1703137. doi:10.1002/aenm.201703137
De Schepper, W., Moraru, M., Jacobs, B., Oudshoorn, M., and Helsen, J. (2019). Electrodialysis of aqueous NaCl-glycerol solutions: a phenomenological comparison of various ion exchange membranes. Sep. Purif. Technol. 217, 274–283. doi:10.1016/j.seppur.2019.02.030
Diallo, M. S., Kotte, M. R., and Cho, M. (2015). Mining critical metals and elements from seawater: opportunities and challenges. Environ. Sci. Technol. 49 (16), 9390–9399. doi:10.1021/acs.est.5b00463
Dock, A., Linders, J., David, M., Gollasch, S., and David, J. (2019). Is human health sufficiently protected from chemicals discharged with treated ballast water from vessels worldwide? A decadal perspective and risk assessment. Chemosphere 235, 194–204. doi:10.1016/j.chemosphere.2019.06.101
DoE, U. (2017). Target explanation document: onboard hydrogen storage for light-duty fuel cell vehicles. U. S. Drive 1, 1–29.
Du, F., Warsinger, D. M., Urmi, T. I., Thiel, G. P., Kumar, A., and Lienhard V, J. H. (2018). Sodium hydroxide production from seawater desalination brine: process design and energy efficiency. Environ. Sci. Technol. 52 (10), 5949–5958. doi:10.1021/acs.est.8b01195
Durbin, D., and Malardier-Jugroot, C. (2013). Review of hydrogen storage techniques for on board vehicle applications. Int. J. hydrogen energy 38 (34), 14595–14617. doi:10.1016/j.ijhydene.2013.07.058
Esselen, G. J., and Bacon, F. S. (1938). Raw materials of the plastic industry. Industrial Eng. Chem. 30 (2), 125–130. doi:10.1021/ie50338a002
Fair, G. M., Morris, J. C., Chang, S. L., Weil, I., and Burden, R. P. (1948). The behavior of chlorine as a water disinfectant. J. AWWA 40 (10), 1051–1061. doi:10.1002/j.1551-8833.1948.tb15055.x
Farha, O. K., Özgür Yazaydın, A., Eryazici, I., Malliakas, C. D., Hauser, B. G., Kanatzidis, M. G., et al. (2010). De novo synthesis of a metal–organic framework material featuring ultrahigh surface area and gas storage capacities. Nat. Chem. 2 (11), 944–948. doi:10.1038/nchem.834
Fertig, M. P., Skadell, K., Schulz, M., Dirksen, C., Adelhelm, P., and Stelter, M. (2022). From high-to low-temperature: the revival of sodium-beta alumina for sodium solid-state batteries. Batter. Supercaps 5 (1), e202100131. doi:10.1002/batt.202100131
GlobeNewswire (2023). Global caustic soda market: analysis by production process, by region size & forecast with impact analysis of COVID-19 and forecast up to 2028. Available at: https://www.globenewswire.com/news-release/2023/04/26/2654786/0/en/Global-Caustic-Soda-Market-Analysis-By-Production-Process-By-Region-Size-Forecast-with-Impact-Analysis-of-COVID-19-and-Forecast-up-to-2028.html.
Go, W., Kim, J., Pyo, J., Wolfenstine, J. B., and Kim, Y. (2021). Investigation on the structure and properties of Na3. 1Zr1. 55Si2. 3P0. 7O11 as a solid electrolyte and its application in a seawater battery. ACS Appl. Mater. Interfaces 13 (44), 52727–52735. doi:10.1021/acsami.1c17338
Go, W., Kim, M.-H., Park, J., Lim, C. H., Joo, S. H., Kim, Y., et al. (2018). Nanocrevasse-rich carbon fibers for stable lithium and sodium metal anodes. Nano Lett. 19 (3), 1504–1511. doi:10.1021/acs.nanolett.8b04106
Goodenough, J. B., Hong, H.-P., and Kafalas, J. (1976). Fast Na+-ion transport in skeleton structures. Mater. Res. Bull. 11 (2), 203–220. doi:10.1016/0025-5408(76)90077-5
Graetz, J. (2009). New approaches to hydrogen storage. Chem. Soc. Rev. 38 (1), 73–82. doi:10.1039/b718842k
Granados Mendoza, P., Moshtarikhah, S., Langenhan, A., de Groot, M., Keurentjes, J., Schouten, J., et al. (2017). Intensification of the chlor-alkali process by using a spinning disc membrane electrolyzer. Chem. Eng. Res. Des. 128, 120–129. doi:10.1016/j.cherd.2017.10.001
Guo, Y., Li, H., and Zhai, T. (2017). Reviving lithium-metal anodes for next-generation high-energy batteries. Adv. Mater. 29 (29), 1700007. doi:10.1002/adma.201700007
Han, J., Hwang, S. M., Go, W., Senthilkumar, S., Jeon, D., and Kim, Y. (2018). Development of coin-type cell and engineering of its compartments for rechargeable seawater batteries. J. Power Sources 374, 24–30. doi:10.1016/j.jpowsour.2017.11.022
Harris, I., Book, D., Anderson, P., and Edwards, P. (2004). Hydrogen storage: the grand challenge. Fuel Cell Rev. 1 (1), 17–23.
He, G., Li, Z., Zhao, J., Wang, S., Wu, H., Guiver, M. D., et al. (2015). Nanostructured ion-exchange membranes for fuel cells: recent advances and perspectives. Adv. Mater. 27 (36), 5280–5295. doi:10.1002/adma.201501406
Hine, F., O'Brien, T. F., and Bommaraju, T. V. (2005). Handbook of chlor-alkali technology: volume I, fundamentals. Berlin, Germany: Springer.
Hong, J. G., Zhang, B., Glabman, S., Uzal, N., Dou, X., Zhang, H., et al. (2015). Potential ion exchange membranes and system performance in reverse electrodialysis for power generation: a review. J. Membr. Sci. 486, 71–88. doi:10.1016/j.memsci.2015.02.039
Hwang, J.-Y., Myung, S.-T., and Sun, Y.-K. (2017). Sodium-ion batteries: present and future. Chem. Soc. Rev. 46 (12), 3529–3614. doi:10.1039/c6cs00776g
Hwang, S. M., Kim, J., Kim, Y., and Kim, Y. (2016). Na-ion storage performance of amorphous Sb 2 S 3 nanoparticles: anode for Na-ion batteries and seawater flow batteries. J. Mater. Chem. A 4 (46), 17946–17951. doi:10.1039/c6ta07838a
Hwang, S. M., Park, J. S., Kim, Y., Go, W., Han, J., Kim, Y., et al. (2019). Rechargeable seawater batteries—from concept to applications. Adv. Mater. 31 (20), 1804936. doi:10.1002/adma.201804936
IEA (2020). “Energy technology perspectives special report on carbon capture utilisation and storage,” in Energy technology perspectives: technical report (Berlin, Germany: Springer), 169.
Izquierdo-Gil, M. A., Barragán, V., Villaluenga, J., and Godino, M. (2012). Water uptake and salt transport through Nafion cation-exchange membranes with different thicknesses. Chem. Eng. Sci. 72, 1–9. doi:10.1016/j.ces.2011.12.040
Jayanthan, S. (2022). Growth of Li-ion battery manufacturing capacity in key EV markets. Available at: https://www.spglobal.com/mobility/en/research-analysis/growth-of-liion-battery-manufacturing-capacity.html (Accessed May 22, 2023).
Kabir, U. (2023). Lithium battery production by country: Top 12 countries. Available at: https://finance.yahoo.com/news/lithium-battery-production-country-top-183050554.html?guccounter=1&guce_referrer=aHR0cHM6Ly93d3cuZ29vZ2xlLmNvbS8&guce_referrer_sig=AQAAAAP1BnkghFlcrwhrs_E26L3q_aRuZyWUSqy8Ku4-UASpGWlAcGLLFzAl-vv1vfSerj9cMlWZ5JeU3pBzwHJGBjQ3qaJaRTd_lzIkrFNn37rZafNHe9OfrAzk_XSQwSiMt6nafJKQdRKbbeRF4XJRJUA6i88VLcXzx24_q3fkSXBG (Accessed September 07, 2023).
Kameche, M., Xu, F., Innocent, C., Pourcelly, G., and Derriche, Z. (2007). Characterisation of Nafion®117 membrane modified chemically with a conducting polymer: an application to the demineralisation of sodium iodide organic solutions. Sep. Purif. Technol. 52 (3), 497–503. doi:10.1016/j.seppur.2006.06.001
Kang, B., Kim, H. J., and Kim, D.-K. (2018). Membrane electrode assembly for energy harvesting from salinity gradient by reverse electrodialysis. J. Membr. Sci. 550, 286–295. doi:10.1016/j.memsci.2018.01.006
Karlsson, R. K., and Cornell, A. (2016). Selectivity between oxygen and chlorine evolution in the chlor-alkali and chlorate processes. Chem. Rev. 116 (5), 2982–3028. doi:10.1021/acs.chemrev.5b00389
Khan, M., Al-Attas, T., Roy, S., Rahman, M. M., Ghaffour, N., Thangadurai, V., et al. (2021). Seawater electrolysis for hydrogen production: a solution looking for a problem? Energy & Environ. Sci. 14 (9), 4831–4839. doi:10.1039/d1ee00870f
Khatun, S., Hirani, H., and Roy, P. (2021). Seawater electrocatalysis: activity and selectivity. J. Mater. Chem. A 9 (1), 74–86. doi:10.1039/d0ta08709b
Kim, D., Park, J.-S., Lee, W.-G., Choi, Y., and Kim, Y. (2022a). Development of rechargeable seawater battery module. J. Electrochem. Soc. 169 (4), 040508. doi:10.1149/1945-7111/ac6142
Kim, D. H., Choi, H., Hwang, D. Y., Park, J., Kim, K. S., Ahn, S., et al. (2018). Reliable seawater battery anode: controlled sodium nucleation via deactivation of the current collector surface. J. Mater. Chem. A 6 (40), 19672–19680. doi:10.1039/c8ta07610c
Kim, H., Park, J.-S., Sahgong, S. H., Park, S., Kim, J.-K., and Kim, Y. (2014a). Metal-free hybrid seawater fuel cell with an ether-based electrolyte. J. Mater. Chem. A 2 (46), 19584–19588. doi:10.1039/c4ta04937c
Kim, J., Park, J., Lee, J., Lim, W. G., Jo, C., and Lee, J. (2021a). Biomass-derived P, N self-doped hard carbon as bifunctional oxygen electrocatalyst and anode material for seawater batteries. Adv. Funct. Mater. 31 (22), 2010882. doi:10.1002/adfm.202010882
Kim, J.-K., Mueller, F., Kim, H., Bresser, D., Park, J.-S., Lim, D.-H., et al. (2014b). Rechargeable-hybrid-seawater fuel cell. NPG Asia Mater. 6 (11), e144. doi:10.1038/am.2014.106
Kim, K., Hwang, S. M., Park, J.-S., Han, J., Kim, J., and Kim, Y. (2016a). Highly improved voltage efficiency of seawater battery by use of chloride ion capturing electrode. J. Power Sources 313, 46–50. doi:10.1016/j.jpowsour.2016.02.060
Kim, N., Jeong, S., Go, W., and Kim, Y. (2022b). A Na+ ion-selective desalination system utilizing a NASICON ceramic membrane. Water Res. 215, 118250. doi:10.1016/j.watres.2022.118250
Kim, N., Park, J.-S., Harzandi, A. M., Kishor, K., Ligaray, M., Cho, K. H., et al. (2020a). Compartmentalized desalination and salination by high energy density desalination seawater battery. Desalination 495, 114666. doi:10.1016/j.desal.2020.114666
Kim, Y., Harzandi, A. M., Lee, J., Choi, Y., and Kim, Y. (2021b). Design of large-scale rectangular cells for rechargeable seawater batteries. Adv. Sustain. Syst. 5 (1), 2000106. doi:10.1002/adsu.202000106
Kim, Y., Hwang, S. M., Yu, H., and Kim, Y. (2018a). High energy density rechargeable metal-free seawater batteries: a phosphorus/carbon composite as a promising anode material. J. Mater. Chem. A 6 (7), 3046–3054. doi:10.1039/c7ta10668h
Kim, Y., Jung, J., Yu, H., Kim, G. T., Jeong, D., Bresser, D., et al. (2020b). Sodium biphenyl as anolyte for sodium–seawater batteries. Adv. Funct. Mater. 30 (24), 2001249. doi:10.1002/adfm.202001249
Kim, Y., Kim, H., Park, S., Seo, I., and Kim, Y. (2016b). Na ion-conducting ceramic as solid electrolyte for rechargeable seawater batteries. Electrochimica Acta 191, 1–7. doi:10.1016/j.electacta.2016.01.054
Kim, Y., Kim, J.-K., Vaalma, C., Bae, G. H., Kim, G.-T., Passerini, S., et al. (2018b). Optimized hard carbon derived from starch for rechargeable seawater batteries. Carbon 129, 564–571. doi:10.1016/j.carbon.2017.12.059
Kim, Y., Shin, K., Jung, Y., Lee, W. G., and Kim, Y. (2022c). Development of prismatic cells for rechargeable seawater batteries. Adv. Sustain. Syst. 6 (6), 2100484. doi:10.1002/adsu.202100484
Kong, H., Yang, M., Miao, Y., and Zhao, X. (2019). Polypyrrole as a novel chloride-storage electrode for seawater desalination. Energy Technol. 7 (11), 1900835. doi:10.1002/ente.201900835
Kumar, A., Du, F., and Lienhard, J. H. (2021). Caustic soda production, energy efficiency, and electrolyzers. ACS Energy Lett. 6 (10), 3563–3566. doi:10.1021/acsenergylett.1c01827
Kurt, C., and Bittner, J. (2000). “Sodium hydroxide,” in Ullmann's encyclopedia of industrial chemistry (United States: Wiley).
Lakshmanan, S., and Murugesan, T. (2014). The chlor-alkali process: work in progress. Clean Technol. Environ. Policy 16, 225–234. doi:10.1007/s10098-013-0630-6
Langmi, H. W., Ren, J., North, B., Mathe, M., and Bessarabov, D. (2014). Hydrogen storage in metal-organic frameworks: a review. Electrochimica Acta 128, 368–392. doi:10.1016/j.electacta.2013.10.190
Lee, C., Jeon, D., Park, J., Lee, W., Park, J., Kang, S. J., et al. (2020a). Tetraruthenium polyoxometalate as an atom-efficient bifunctional oxygen evolution reaction/oxygen reduction reaction catalyst and its application in seawater batteries. ACS Appl. Mater. interfaces 12 (29), 32689–32697. doi:10.1021/acsami.0c07225
Lee, W., Park, J., Park, J., Kang, S. J., Choi, Y., and Kim, Y. (2020b). Identifying the mechanism and impact of parasitic reactions occurring in carbonaceous seawater battery cathodes. J. Mater. Chem. A 8 (18), 9185–9193. doi:10.1039/d0ta02913k
Ligaray, M., Kim, N., Park, S., Park, J.-S., Park, J., Kim, Y., et al. (2020). Energy projection of the seawater battery desalination system using the reverse osmosis system analysis model. Chem. Eng. J. 395, 125082. doi:10.1016/j.cej.2020.125082
Lim, D.-H., Dong, C., Kim, H., Bae, G.-H., Choo, K., Cho, G.-B., et al. (2021). Redox chemistry of advanced functional material for low-cost and environment-friendly seawater energy storage. Mater. Today Energy 21, 100805. doi:10.1016/j.mtener.2021.100805
Lim, K. L., Kazemian, H., Yaakob, Z., and Daud, W. R. W. (2010). Solid-state materials and methods for hydrogen storage: a critical review. Chem. Eng. Technol. 33 (2), 213–226. doi:10.1002/ceat.200900376
Linkov, V., and Belyakov, V. (2001). Novel ceramic membranes for electrodialysis. Sep. Purif. Technol. 25 (1-3), 57–63. doi:10.1016/s1383-5866(01)00090-9
Liu, G., Xu, Y., Yang, T., and Jiang, L. (2023). Recent advances in electrocatalysts for seawater splitting. Nano Mater. Sci. 5, 101–116. doi:10.1016/j.nanoms.2020.12.003
Liu, J., Duan, S., Shi, H., Wang, T., Yang, X., Huang, Y., et al. (2022). Rationally designing efficient electrocatalysts for direct seawater splitting: challenges, achievements, and promises. Angewandte Chemie Int. ed. Engl. 61 (45), e202210753. doi:10.1002/anie.202210753
Loganathan, P., Naidu, G., and Vigneswaran, S. (2017). Mining valuable minerals from seawater: a critical review. Environ. Sci. Water Res. Technol. 3 (1), 37–53. doi:10.1039/c6ew00268d
Lu, L., Lu, Y., Alonso, J. A., López, C. A., Fernández-Díaz, M. T., Zou, B., et al. (2021). A monolithic solid-state sodium–sulfur battery with Al-doped Na3. 4Zr2 (Si0. 8P0. 2O4) 3 electrolyte. ACS Appl. Mater. Interfaces 13 (36), 42927–42934. doi:10.1021/acsami.1c13000
Lu, Y., Li, L., Zhang, Q., Niu, Z., and Chen, J. (2018). Electrolyte and interface engineering for solid-state sodium batteries. Joule 2 (9), 1747–1770. doi:10.1016/j.joule.2018.07.028
Ma, Q., Guin, M., Naqash, S., Tsai, C.-L., Tietz, F., and Guillon, O. (2016). Scandium-substituted Na3Zr2 (SiO4) 2 (PO4) prepared by a solution-assisted solid-state reaction method as sodium-ion conductors. Chem. Mater. 28 (13), 4821–4828. doi:10.1021/acs.chemmater.6b02059
Marleen Pauwels, J. V. D. B. (2023). Chlor-alkali industry review: remaining safe and competitive while achieving climate neutrality. United States: Euro Chlor.
Martin, G., Rentsch, L., Höck, M., and Bertau, M. (2017). Lithium market research–global supply, future demand and price development. Energy Storage Mater. 6, 171–179. doi:10.1016/j.ensm.2016.11.004
Matin, A., Khan, Z., Zaidi, S., and Boyce, M. (2011). Biofouling in reverse osmosis membranes for seawater desalination: phenomena and prevention. Desalination 281, 1–16. doi:10.1016/j.desal.2011.06.063
Matteson, S., and Williams, E. (2015). Residual learning rates in lead-acid batteries: effects on emerging technologies. Energy Policy 85, 71–79. doi:10.1016/j.enpol.2015.05.014
Mauguin, G., and Corsin, P. (2005). Concentrate and other waste disposals from SWRO plants: characterization and reduction of their environmental impact. Desalination 182 (1-3), 355–364. doi:10.1016/j.desal.2005.02.033
Momirlan, M., and Veziroglu, T. (2002). Current status of hydrogen energy. Renew. Sustain. energy Rev. 6 (1-2), 141–179. doi:10.1016/s1364-0321(02)00004-7
Moradi, R., and Groth, K. M. (2019). Hydrogen storage and delivery: review of the state of the art technologies and risk and reliability analysis. Int. J. Hydrogen Energy 44 (23), 12254–12269. doi:10.1016/j.ijhydene.2019.03.041
Nam, D.-H., and Choi, K.-S. (2017). Bismuth as a new chloride-storage electrode enabling the construction of a practical high capacity desalination battery. J. Am. Chem. Soc. 139 (32), 11055–11063. doi:10.1021/jacs.7b01119
O'Brien, T. F., Bommaraju, T. V., and Hine, F. (2005). Handbook of chlor-alkali technology: volume I: fundamentals, volume II: brine treatment and cell operation, volume III: facility design and product handling, volume IV: plant commissioning and support systems, volume V: corrosion, environmental issues, and future development. Berlin, Germany: Springer.
Oh, J. A. S., He, L., Plewa, A., Morita, M., Zhao, Y., Sakamoto, T., et al. (2019). Composite NASICON (Na3Zr2Si2PO12) solid-state electrolyte with enhanced Na+ ionic conductivity: effect of liquid phase sintering. ACS Appl. Mater. Interfaces 11 (43), 40125–40133. doi:10.1021/acsami.9b14986
Pan, L., Zhang, X., Yang, M., Han, J., Jiang, J., Li, W., et al. (2019). Effects of dechlorination conditions on the developmental toxicity of a chlorinated saline primary sewage effluent: excessive dechlorination is better than not enough. Sci. Total Environ. 692, 117–126. doi:10.1016/j.scitotenv.2019.07.207
Panagopoulos, A. (2020). Process simulation and techno-economic assessment of a zero liquid discharge/multi-effect desalination/thermal vapor compression (ZLD/MED/TVC) system. Int. J. Energy Res. 44 (1), 473–495. doi:10.1002/er.4948
Panagopoulos, A. (2021). Techno-economic assessment of minimal liquid discharge (MLD) treatment systems for saline wastewater (brine) management and treatment. Process Saf. Environ. Prot. 146, 656–669. doi:10.1016/j.psep.2020.12.007
Panagopoulos, A. (2022). Techno-economic assessment of zero liquid discharge (ZLD) systems for sustainable treatment, minimization and valorization of seawater brine. J. Environ. Manag. 306, 114488. doi:10.1016/j.jenvman.2022.114488
Panagopoulos, A., Haralambous, K.-J., and Loizidou, M. (2019). Desalination brine disposal methods and treatment technologies-A review. Sci. Total Environ. 693, 133545. doi:10.1016/j.scitotenv.2019.07.351
Park, J., Park, J.-S., Senthilkumar, S., and Kim, Y. (2020). Hybridization of cathode electrochemistry in a rechargeable seawater battery: toward performance enhancement. J. Power Sources 450, 227600. doi:10.1016/j.jpowsour.2019.227600
Park, J.-S., Kim, S., Choi, Y. M., M Harzandi, A., and Kim, Y. (2021). Disinfection-dechlorination battery for safe water production. ACS ES&T Water 1 (9), 2146–2154. doi:10.1021/acsestwater.1c00207
Park, S., Kim, N., Kim, Y., Son, M., and Cho, K. H. (2022). Seawater battery desalination with a reverse osmosis membrane for simultaneous brine treatment and energy storage. J. Clean. Prod. 333, 130188. doi:10.1016/j.jclepro.2021.130188
Park, S., SenthilKumar, B., Kim, K., Hwang, S. M., and Kim, Y. (2016). Saltwater as the energy source for low-cost, safe rechargeable batteries. J. Mater. Chem. A 4 (19), 7207–7213. doi:10.1039/c6ta01274d
Preuster, P., Alekseev, A., and Wasserscheid, P. (2017). Hydrogen storage technologies for future energy systems. Annu. Rev. Chem. Biomol. Eng. 8, 445–471. doi:10.1146/annurev-chembioeng-060816-101334
Qiu, Y., Liu, Z., Sun, A., Zhang, X., Ji, X., and Liu, J. (2022). Electrochemical in situ self-healing of porous nanosheets based on the phase reconstruction of carbonate hydroxide to layered double hydroxides with unsaturated coordination metal sites for high-performance water oxidation. ACS Sustain. Chem. Eng. 10 (49), 16417–16426. doi:10.1021/acssuschemeng.2c05705
Qiu, Y., Lv, Y., Tang, C., Liao, J., Ruan, H., Sotto, A., et al. (2021). Sustainable recovery of high-saline papermaking wastewater: optimized separation for salts and organics via membrane-hybrid process. Desalination 507, 114938. doi:10.1016/j.desal.2021.114938
Rao, Y. B., Bharathi, K. K., and Patro, L. (2021). Review on the synthesis and doping strategies in enhancing the Na ion conductivity of Na3Zr2Si2PO12 (NASICON) based solid electrolytes. Solid State Ionics 366-367, 115671. doi:10.1016/j.ssi.2021.115671
Reig, M., Casas, S., Aladjem, C., Valderrama, C., Gibert, O., Valero, F., et al. (2014). Concentration of NaCl from seawater reverse osmosis brines for the chlor-alkali industry by electrodialysis. Desalination 342, 107–117. doi:10.1016/j.desal.2013.12.021
Ren, J., Musyoka, N. M., Langmi, H. W., Mathe, M., and Liao, S. (2017). Current research trends and perspectives on materials-based hydrogen storage solutions: a critical review. Int. J. hydrogen energy 42 (1), 289–311. doi:10.1016/j.ijhydene.2016.11.195
Rivard, E., Trudeau, M., and Zaghib, K. (2019). Hydrogen storage for mobility: a review. Materials 12 (12), 1973. doi:10.3390/ma12121973
Roberts, D. A., Johnston, E. L., and Knott, N. A. (2010). Impacts of desalination plant discharges on the marine environment: a critical review of published studies. Water Res. 44 (18), 5117–5128. doi:10.1016/j.watres.2010.04.036
Ronquim, F. M., Sakamoto, H. M., Mierzwa, J. C., Kulay, L., and Seckler, M. M. (2020). Eco-efficiency analysis of desalination by precipitation integrated with reverse osmosis for zero liquid discharge in oil refineries. J. Clean. Prod. 250, 119547. doi:10.1016/j.jclepro.2019.119547
Rosen, M. A., and Koohi-Fayegh, S. (2016). The prospects for hydrogen as an energy carrier: an overview of hydrogen energy and hydrogen energy systems. Energy, Ecol. Environ. 1, 10–29. doi:10.1007/s40974-016-0005-z
Ryu, J. H., Park, J., Park, J., Mun, J., Im, E., Lee, H., et al. (2022). Carbothermal shock-induced bifunctional Pt-Co alloy electrocatalysts for high-performance seawater batteries. Energy Storage Mater. 45, 281–290. doi:10.1016/j.ensm.2021.11.036
Sadaoka, Y. (2007). NASICON based CO2 gas sensor with an auxiliary electrode composed of LiCO3-metal oxide mixtures. Sensors Actuators B Chem. 121 (1), 194–199. doi:10.1016/j.snb.2006.09.019
Schlapbach, L., and Züttel, A. (2001). Hydrogen-storage materials for mobile applications. nature 414 (6861), 353–358. doi:10.1038/35104634
Sedivy, V. M. (2008). Economy of salt in chloralkali manufacture, in National Salt Conference, Gandhidham, India, 25 April, 2008 Springer), 1–9.
Selvaraj, H., Aravind, P., and Sundaram, M. (2018). Four compartment mono selective electrodialysis for separation of sodium formate from industry wastewater. Chem. Eng. J. 333, 162–169. doi:10.1016/j.cej.2017.09.150
Senthilkumar, S., Abirami, M., Kim, J., Go, W., Hwang, S. M., and Kim, Y. (2017). Sodium-ion hybrid electrolyte battery for sustainable energy storage applications. J. Power Sources 341, 404–410. doi:10.1016/j.jpowsour.2016.12.015
Senthilkumar, S., Han, J., Park, J., Min Hwang, S., Jeon, D., and Kim, Y. (2018). Energy efficient Na-aqueous-catholyte redox flow battery. Energy Storage Mater. 12, 324–330. doi:10.1016/j.ensm.2017.10.006
Sharma, P., Han, J., Park, J., Kim, D. Y., Lee, J., Oh, D., et al. (2021). Alkali-metal-mediated reversible chemical hydrogen storage using seawater. JACS Au 1 (12), 2339–2348. doi:10.1021/jacsau.1c00444
Shen, L., Yang, J., Liu, G., Avdeev, M., and Yao, X. (2021). High ionic conductivity and dendrite-resistant NASICON solid electrolyte for all-solid-state sodium batteries. Mater. Today Energy 20, 100691. doi:10.1016/j.mtener.2021.100691
Shin, K. H., Park, J., Park, S. K., Nakhanivej, P., Hwang, S. M., Kim, Y., et al. (2019). Cobalt vanadate nanoparticles as bifunctional oxygen electrocatalysts for rechargeable seawater batteries. J. Industrial Eng. Chem. 72, 250–254. doi:10.1016/j.jiec.2018.12.025
Slater, M. D., Kim, D., Lee, E., and Johnson, C. S. (2013). Sodium-ion batteries. Adv. Funct. Mater. 23 (8), 947–958. doi:10.1002/adfm.201200691
Son, M., Park, S., Kim, N., Angeles, A. T, Kim, Y., and Cho, K. H. (2021). Simultaneous energy storage and seawater desalination using rechargeable seawater battery:Feasibility and future directions. Advanced Science 8 (18), 2101289.
Son, M., Shim, J., Park, S., Yoon, N., Jeong, K., and Cho, K. H. (2022). Seawater battery desalination with sodium-intercalation cathode for hypersaline water treatment. Desalination 531, 115713. doi:10.1016/j.desal.2022.115713
Song, S., Duong, H. M., Korsunsky, A. M., Hu, N., and Lu, L. (2016). A Na+ superionic conductor for room-temperature sodium batteries. Sci. Rep. 6 (1), 32330. doi:10.1038/srep32330
Statista (2023). Market volume of chlorine worldwide from 2015 to 2022, with a forecast for 2023 to 2030. Mumbai: AgileIntel Research.
Sugiyama, M., Saiki, K., Sakata, A., Aikawa, H., and Furuya, N. (2003). Accelerated degradation testing of gas diffusion electrodes for the chlor-alkali process. J. Appl. Electrochem. 33, 929–932. doi:10.1023/a:1025899204203
Tarasov, B., Lototskii, M., and Yartys’, V. (2007). Problem of hydrogen storage and prospective uses of hydrides for hydrogen accumulation. Russ. J. general Chem. 77, 694–711. doi:10.1134/s1070363207040329
The Essential Chemical Industry (2018). Sodium hydroxide. The essential chemical industry. Available at: https://www.essentialchemicalindustry.org/chemicals/sodium-hydroxide.html (Accessed September 13, 2023).
Usman, M. R. (2022). Hydrogen storage methods: review and current status. Renew. Sustain. Energy Rev. 167, 112743. doi:10.1016/j.rser.2022.112743
Wentker, M., Greenwood, M., and Leker, J. (2019). A bottom-up approach to lithium-ion battery cost modeling with a focus on cathode active materials. Energies 12 (3), 504. doi:10.3390/en12030504
Wi, T.-U., Lee, C., Rahman, M. F., Go, W., Kim, S. H., Hwang, D. Y., et al. (2020). Chemical stability and degradation mechanism of solid electrolytes/aqueous media at a steady state for long-lasting sodium batteries. Chem. Mater. 33 (1), 126–135. doi:10.1021/acs.chemmater.0c03022
Wolfenstine, J., Go, W., Kim, Y., and Sakamoto, J. (2023). Mechanical properties of NaSICON: a brief review. Ionics 29 (1), 1–8. doi:10.1007/s11581-022-04820-z
WolframAlpha (2014). Crust abundance. Available at: https://www.wolframalpha.com/input?i=%22CrustAbundance%22 (Accessed September 13, 2023).
Xi, X.-l., Feng, M., Zhang, L.-w., and Nie, Z.-r. (2020). Applications of molten salt and progress of molten salt electrolysis in secondary metal resource recovery. Int. J. Minerals, Metallurgy Mater. 27, 1599–1617. doi:10.1007/s12613-020-2175-0
Yang, C., Dong, K., Zhang, L., He, X., Chen, J., Sun, S., et al. (2023). Improved alkaline seawater splitting of NiS nanosheets by iron doping. Inorg. Chem. 62 (20), 7976–7981. doi:10.1021/acs.inorgchem.3c00836
Yang, C., Fu, K., Zhang, Y., Hitz, E., and Hu, L. (2017). Protected lithium-metal anodes in batteries: from liquid to solid. Adv. Mater. 29 (36), 1701169. doi:10.1002/adma.201701169
Yang, Z., Tang, B., Xie, Z., and Zhou, Z. (2021). NASICON-type Na3Zr2Si2PO12 solid-state electrolytes for sodium batteries. ChemElectroChem 8 (6), 1035–1047. doi:10.1002/celc.202001527
Youme (2022). Top 10 lithium ion battery separator company in China. Available at: https://youmecs.com/2022-top-10-lithium-ion-battery-separator-company/ (Accessed September 13, 2023).
Yu, M., Li, J., Liu, F., Liu, J., Xu, W., Hu, H., et al. (2022). Anionic formulation of electrolyte additive towards stable electrocatalytic oxygen evolution in seawater splitting. J. Energy Chem. 72, 361–369. doi:10.1016/j.jechem.2022.04.004
Zhang, F., Zhao, P., Niu, M., and Maddy, J. (2016). The survey of key technologies in hydrogen energy storage. Int. J. hydrogen energy 41 (33), 14535–14552. doi:10.1016/j.ijhydene.2016.05.293
Zhang, L., Yasin, A., Li, M., Hao, B., and Ma, P.-C. (2021). Silicate based solar evaporator with self-cleaning and corrosion resistant properties for durable seawater desalination. Sustain. Mater. Technol. 30, e00362. doi:10.1016/j.susmat.2021.e00362
Zhang, W., Miao, M., Pan, J., Sotto, A., Shen, J., Gao, C., et al. (2017). Separation of divalent ions from seawater concentrate to enhance the purity of coarse salt by electrodialysis with monovalent-selective membranes. Desalination 411, 28–37. doi:10.1016/j.desal.2017.02.008
Zhang, Y., Park, J.-S., Senthilkumar, S. T., and Kim, Y. (2018). A novel rechargeable hybrid Na-seawater flow battery using bifunctional electrocatalytic carbon sponge as cathode current collector. J. Power Sources 400, 478–484. doi:10.1016/j.jpowsour.2018.08.044
Zhao, C., Liu, L., Qi, X., Lu, Y., Wu, F., Zhao, J., et al. (2018). Solid-state sodium batteries. Adv. Energy Mater. 8 (17), 1703012. doi:10.1002/aenm.201703012
Zhao, K., Bkour, Q., Kim, B. H., Norton, M. G., and Ha, S. (2019). Front cover: NiMo-Ceria-Zirconia catalyst for inert-substrate-supported tubular solid oxide fuel cells running on model gasoline (energy technol. 1/2019). Energy Technol. 7 (1), 1. doi:10.1002/ente.201980101
Zhao, T., Ji, X., Jin, W., Yang, W., and Li, T. (2017). Hydrogen storage capacity of single-walled carbon nanotube prepared by a modified arc discharge. Fullerenes, Nanotub. Carbon Nanostructures 25 (6), 355–358. doi:10.1080/1536383x.2017.1305358
Zheng, X., Bommier, C., Luo, W., Jiang, L., Hao, Y., and Huang, Y. (2019). Sodium metal anodes for room-temperature sodium-ion batteries: applications, challenges and solutions. Energy Storage Mater. 16, 6–23. doi:10.1016/j.ensm.2018.04.014
Zhou, D., Zhu, L., Fu, Y., Zhu, M., and Xue, L. (2015). Development of lower cost seawater desalination processes using nanofiltration technologies—a review. Desalination 376, 109–116. doi:10.1016/j.desal.2015.08.020
Keywords: NASICON, seawater, seawater battery, hydrogen production, NaOH production, chlorine production, seawater desalination, mineral extraction
Citation: Kim N, Kim S, Jeong S, Jin H, Lee H, Kim Y, Lee W-G and Park J-S (2023) Seawater to resource technologies with NASICON solid electrolyte: a review. Front. Batteries Electrochem. 2:1301806. doi: 10.3389/fbael.2023.1301806
Received: 25 September 2023; Accepted: 02 November 2023;
Published: 21 November 2023.
Edited by:
Jung Ho Kim, University of Wollongong, AustraliaReviewed by:
Junyoung Mun, Sungkyunkwan University, Republic of KoreaGuosheng Li, Pacific Northwest National Laboratory (DOE), United States
Copyright © 2023 Kim, Kim, Jeong, Jin, Lee, Kim, Lee and Park. This is an open-access article distributed under the terms of the Creative Commons Attribution License (CC BY). The use, distribution or reproduction in other forums is permitted, provided the original author(s) and the copyright owner(s) are credited and that the original publication in this journal is cited, in accordance with accepted academic practice. No use, distribution or reproduction is permitted which does not comply with these terms.
*Correspondence: Jeong-Sun Park, jspark9191@gmail.com; Wang-Geun Lee, wglee8786@unist.ac.kr